10 Magnetism
Learning Objectives
After successful completion of this section, you will be able to demonstrate competency in the following areas:
- Define magnetic force (CLO1)(CLO2)(CLO3)
- Explain the effects of magnetic fields on moving charge (CLO2)(CLO3)
- Describe how electric motors work and the role of magnetism in their function (CLO2)(CLO3)
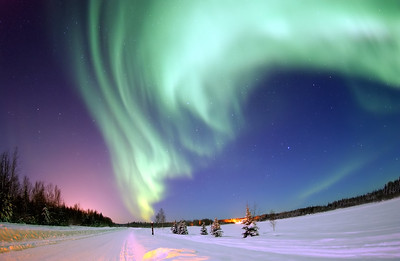
Introduction to Magnetism
One evening, an Alaskan sticks a note to his refrigerator with a small magnet. Through the kitchen window, the aurora borealis glows in the night sky. This grand spectacle is shaped by the same force that holds the note to the refrigerator.
People have been aware of magnets and magnetism for thousands of years. The earliest records date to well before the time of Christ, particularly in a region of Asia Minor called Magnesia (the name of this region is the source of words like magnetic). Magnetic rocks found in Magnesia, which is now part of western Turkey, stimulated interest during ancient times. A practical application for magnets was found later, when they were employed as navigational compasses. The use of magnets in compasses resulted not only in improved long-distance sailing, but also in the names of “north” and “south” being given to the two types of magnetic poles.
Today magnetism plays many important roles in our lives. Physicists’ understanding of magnetism has enabled the development of technologies that affect our everyday lives. The iPhone in your purse or pocket, for example, wouldn’t have been possible without the applications of magnetism and electricity on a small scale.
All electric motors, with uses as diverse as powering refrigerators, starting cars, and moving elevators, contain magnets. Generators, whether producing hydroelectric power or running bicycle lights, use magnetic fields. Recycling facilities employ magnets to separate iron from other refuse. Hundreds of millions of dollars are spent annually on magnetic containment of fusion as a future energy source. Magnetic resonance imaging (MRI) has become an important diagnostic tool in the field of medicine, and the use of magnetism to explore brain activity is a subject of contemporary research and development. The list of applications also includes computer hard drives, tape recording, detection of inhaled asbestos, and levitation of high-speed trains. Magnetism is used to explain atomic energy levels, cosmic rays, and charged particles trapped in the Van Allen belts. Once again, we will find all these disparate phenomena are linked by a small number of underlying physical principles.
Magnets
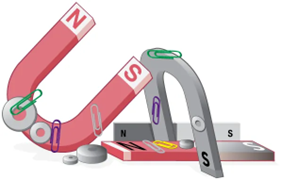
All magnets attract iron, such as that in a refrigerator door. However, magnets may attract or repel other magnets. Experimentation shows that all magnets have two poles. If freely suspended, one pole will point toward the north. The two poles are thus named the north magnetic pole and the south magnetic pole (or more properly, north-seeking and south-seeking poles, for the attractions in those directions).
It is a universal characteristic of all magnets that like poles repel and unlike poles attract. (Note the similarity with electrostatics: unlike charges attract and like charges repel.) Further experimentation shows that it is impossible to separate north and south poles in the manner that + and − charges can be separated.
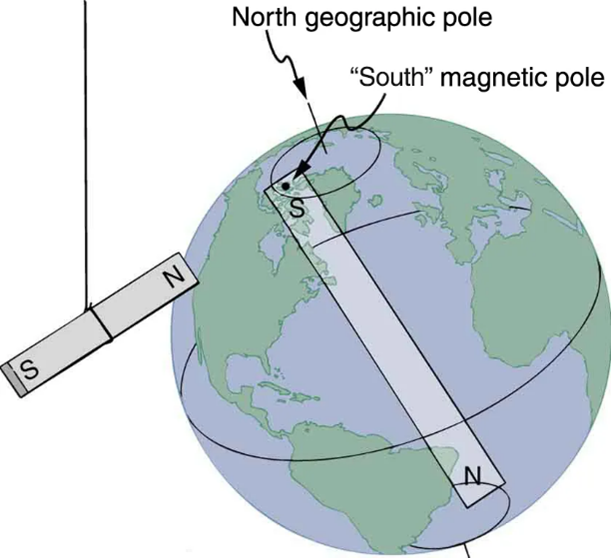
Misconception Alert
Earth acts like a very large bar magnet with its south-seeking pole near the geographic North Pole. That is why the north pole of your compass is attracted toward the geographic north pole of Earth—because the magnetic pole that is near the geographic North Pole is actually a south magnetic pole! Confusion arises because the geographic term “North Pole” has come to be used (incorrectly) for the magnetic pole that is near the North Pole. Thus, “north magnetic pole” is actually a misnomer—it should be called the south magnetic pole.
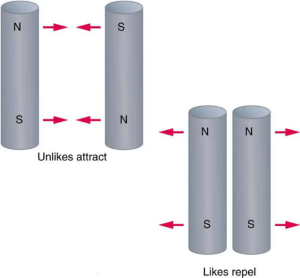
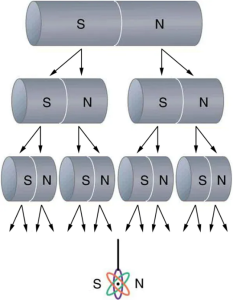
The fact that magnetic poles always occur in pairs of north and south is true from a very large scale—for example, sunspots always occur in pairs that are north and south magnetic poles—all the way down to a very small scale. Magnetic atoms have both a north pole and a south pole, as do many types of subatomic particles, such as electrons, protons, and neutrons.
Take-Home Experiment: Refrigerator Magnets
We know that like magnetic poles repel and unlike poles attract. See if you can show this for two refrigerator magnets. Will the magnets stick if you turn them over? Why do they stick to the door anyway? What can you say about the magnetic properties of the door next to the magnet? Do refrigerator magnets stick to metal or plastic spoons? Do they stick to all types of metal?
Ferromagnets
Only certain materials, such as iron, cobalt, nickel, and gadolinium, exhibit strong magnetic effects. Such materials are called ferromagnetic, after the Latin word for iron, ferrum. A group of materials made from the alloys of the rare earth elements are also used as strong and permanent magnets; a popular one is neodymium. Other materials exhibit weak magnetic effects, which are detectable only with sensitive instruments. Not only do ferromagnets respond strongly to magnets (the way iron is attracted to magnets); they can also be magnetized themselves—that is, they can be induced to be magnetic or made into permanent magnets.
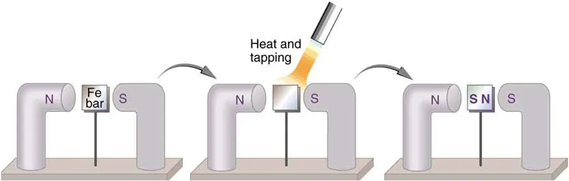
Electromagnets
Early in the 19th century, it was discovered that electrical currents cause magnetic effects. The first significant observation was by the Danish scientist Hans Christian Oersted (1777–1851), who found that a compass needle was deflected by a current-carrying wire. This was the first significant evidence that the movement of charges had any connection with magnets. Electromagnetism is the use of electric current to make magnets. Such a temporarily induced magnet is called an electromagnet. Electromagnets are employed for everything from a wrecking yard crane that lifts scrapped cars to the controls for the beam of a 90-km-circumference particle accelerator to the magnets in medical imaging machines. (See Figure 10.7.)
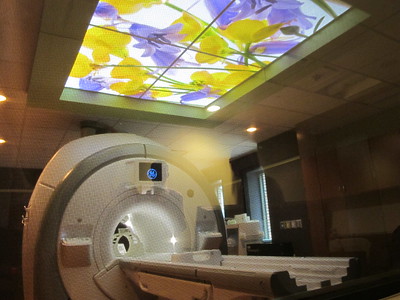
Figure 10.8 shows the response of iron filings to a current-carrying coil and to a permanent bar magnet. The patterns are similar. In fact, electromagnets and ferromagnets have the same basic characteristics—for example, they have north and south poles that cannot be separated and for which like poles repel and unlike poles attract.
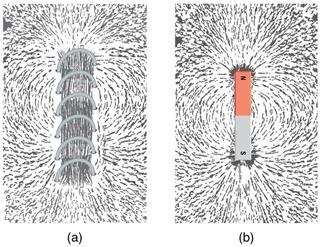
Combining a ferromagnet with an electromagnet can produce particularly strong magnetic effects. (See Figure 10.9.) Whenever strong magnetic effects are needed, such as lifting scrap metal, or in particle accelerators, electromagnets are enhanced by ferromagnetic materials.
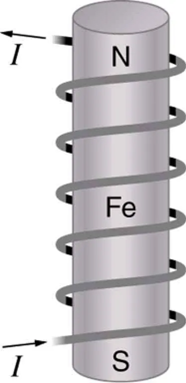
Figure 10.10 shows a few uses of combinations of electromagnets and ferromagnets. Ferromagnetic materials can act as memory devices, because the orientation of the magnetic fields of small domains can be reversed or erased. Magnetic information storage on videotapes and computer hard drives is among the most common applications. This property is vital in our digital world.
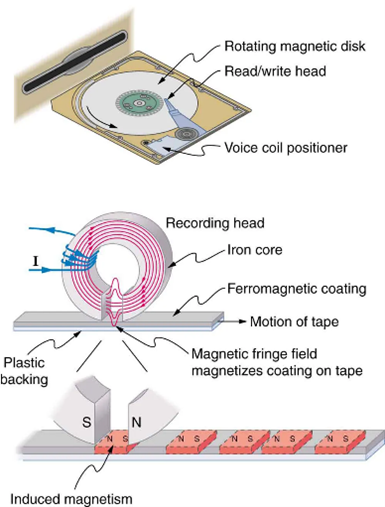
Current: Source of All Magnetism
An electromagnet creates magnetism with an electric current. But what about ferromagnets? Figure 10.12 shows models of how electric currents create magnetism at the submicroscopic level. (Note that we cannot directly observe the paths of individual electrons about atoms, and so a model or visual image, consistent with all direct observations, is made. We can directly observe the electron’s orbital angular momentum, its spin momentum, and subsequent magnetic moments, all of which are explained with electric-current-creating subatomic magnetism.) Currents, including those associated with other submicroscopic particles like protons, allow us to explain ferromagnetism and all other magnetic effects. Ferromagnetism, for example, results from an internal cooperative alignment of electron spins, possible in some materials but not in others.
Crucial to the statement that electric current is the source of all magnetism is the fact that it is impossible to separate north and south magnetic poles. (This is far different from positive and negative charges, which are easily separated.) A current loop always produces a magnetic dipole—that is, a magnetic field that acts like a north pole and south pole pair. Since isolated north and south magnetic poles, called magnetic monopoles, are not observed, currents are used to explain all magnetic effects. If magnetic monopoles did exist, then we would have to modify this underlying connection that all magnetism is due to electrical current. There is no known reason that magnetic monopoles should not exist—they are simply never observed—and so searches at the subnuclear level continue. If they do not exist, we would like to find out why not. If they do exist, we would like to see evidence of them.
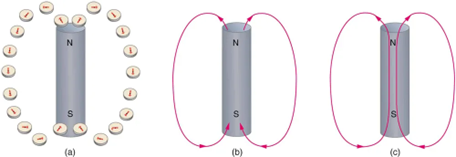
Magnetic Fields and Magnetic Field Lines
Einstein is said to have been fascinated by a compass as a child, perhaps musing on how the needle felt a force without direct physical contact. His ability to think deeply and clearly about action at a distance, particularly for gravitational, electric, and magnetic forces, later enabled him to create his revolutionary theory of relativity. Since magnetic forces act at a distance, we define a magnetic field to represent magnetic forces. The pictorial representation of magnetic field lines is very useful in visualizing the strength and direction of the magnetic field. As shown in Figure 10.12, the direction of magnetic field lines is defined to be the direction in which the north end of a compass needle points. The magnetic field is traditionally called the B-field.
Small compasses used to test a magnetic field will not disturb it. (This is analogous to the way we tested electric fields with a small test charge. In both cases, the fields represent only the object creating them and not the probe testing them.) Figure 10.13 shows how the magnetic field appears for a current loop and a long straight wire, as could be explored with small compasses. A small compass placed in these fields will align itself parallel to the field line at its location, with its north pole pointing in the direction of B. Note the symbols used for field into and out of the paper.
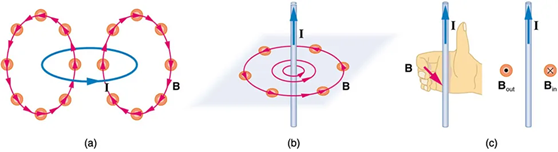
Making Connections: Concept of a Field
A field is a way of mapping forces surrounding any object that can act on another object at a distance without apparent physical connection. The field represents the object generating it. Gravitational fields map gravitational forces, electric fields map electrical forces, and magnetic fields map magnetic forces.
Magnetic Field Rules
Extensive exploration of magnetic fields has revealed a number of hard-and-fast rules. We use magnetic field lines to represent the field (the lines are a pictorial tool, not a physical entity in and of themselves). The properties of magnetic field lines can be summarized by these rules:
- The direction of the magnetic field is tangent to the field line at any point in space. A small compass will point in the direction of the field line.
- The strength of the field is proportional to the closeness of the lines. It is exactly proportional to the number of lines per unit area perpendicular to the lines (called the areal density).
- Magnetic field lines can never cross, meaning that the field is unique at any point in space.
- Magnetic field lines are continuous, forming closed loops without beginning or end. They go from the north pole to the south pole.
The last property is related to the fact that the north and south poles cannot be separated. This is a distinct difference from electric field lines, which begin and end on the positive and negative charges. If magnetic monopoles existed, then magnetic field lines would begin and end on them.
Check Your Understanding
The content in this chapter is adapted from multiple sources. The overall chapter structure is adapted from Exploring the Physical World and used under a Creative Commons Attribution-NonCommercial-ShareAlike 4.0 International License. The sections on introduction to magnetism, ferromagnets, electromagnets, current as the source of magnetism, and magnetic fields are adapted from College Physics 2e by OpenStax and are used under a Creative Commons-Attribution 4.0 International License. Other content and interactive activities are the original work of the authors of this text and are shared under a Creative Commons-Attribution 4.0 International License.
Media Attributions
- Fig. 10.1 © Joshua Strang, USAF is licensed under a CC BY (Attribution) license
- Fig. 10.2 © OpenStax is licensed under a CC BY (Attribution) license
- Fig. 10.3 © OpenStax is licensed under a CC BY (Attribution) license
- Fig. 10.4 © OpenStax is licensed under a CC BY (Attribution) license
- Fig. 10.5 © OpenStax is licensed under a CC BY (Attribution) license
- Fig. 10.6 © OpenStax is licensed under a CC BY (Attribution) license
- Fig. 10.7 © Bill McChesney is licensed under a CC BY (Attribution) license
- Fig. 10.8 © OpenStax is licensed under a CC BY (Attribution) license
- Fig. 10.9 © OpenStax is licensed under a CC BY (Attribution) license
- Fig. 10.10 © OpenStax is licensed under a CC BY (Attribution) license
- Fig. 10.12 © OpenStax is licensed under a CC BY (Attribution) license
- Fig. 10.13 © OpenStax is licensed under a CC BY (Attribution) license
phenomenon associated with magnetic fields, which arise from the motion of electric charges
end or the side of a magnet that is attracted toward Earth’s geographic north pole
end or the side of a magnet that is attracted toward Earth’s geographic south pole
materials, such as iron, cobalt, nickel, and gadolinium, that exhibit strong magnetic effects
to be turned into a magnet; to be induced to be magnetic
use of electrical currents to induce magnetism
object that is temporarily magnetic when an electrical current is passed through it
rate at which charge flows
negatively charged subatomic particles; together with protons and neutrons they compose all atoms
positively charged particles that, together with the electrically neutral particles called neutrons, make up the nucleus of an atom
isolated magnetic poles; a south pole without a north pole, or vice versa (no magnetic monopole has ever been observed)
region around a magnetic material or a moving electric charge within which the force of magnetism acts
pictorial representations of the strength and the direction of magnetic fields
region around a charged particle or object within which a force would be exerted on other charged particles or objects