Chapter 15. The Nervous System
Sharon Lagarde
Unit Outline
Part 1: The Anatomical and Functional Organization of the Nervous System
Part 3: The Central Nervous System
- The Cerebrum
- The Diencephalon
- The Brain Stem
- The Cerebellum
- The Spinal Cord
- The Meninges
- The Ventricular System and Cerebrospinal Fluid Circulation
Learning Objectives
At the end of this unit, you should be able to:
I. Describe the organization of the nervous system and explain the functions of its principal components.
II. Describe the structure of the following: neuron, glia, ganglion, nerve, gray matter, tract, white matter, sensory neuron, motor neuron.
III. Name, locate, and describe the functions of the main areas of the human brain.
IV. Describe the structure and functions of the spinal cord.
V. Describe the components of a reflex arc and explain how a reflex arc works.
VI. Describe the function of the autonomic nervous system (ANS) and compare the specific functions of the parasympathetic and sympathetic divisions of the ANS.
VII. Describe the resting membrane potential of a neuron and explain how it is maintained.
VIII. Explain how a neuronal action potential is generated.
IX. Explain how neuronal action potentials propagate down the axon.
X. Explain the process of neurotransmission and name three different neurotransmitters.
Part 1: Anatomical and Functional Organization of the Nervous System
The picture you have in your mind of the nervous system probably includes the brain, the nervous tissue contained within the cranium, and the spinal cord, the extension of nervous tissue within the vertebral column. But the nervous system is a very complex structure of billions of interacting nerves. Within the brain, many different and separate regions are responsible for many different and separate functions. It is as if the nervous system is composed of many organs that all look similar and can only be differentiated using techniques such as microscopy or electrophysiology. In comparison, it is easy to see that the stomach is different than the esophagus or the liver, so you can imagine the digestive system as a collection of specific organs.
Anatomical Divisions
The nervous system can be divided into two major regions: the central and peripheral nervous systems. The central nervous system (CNS) is the brain and spinal cord, and the peripheral nervous system (PNS) is everything else (Figures 15.1 and 15.2). The brain is contained within the cranial cavity of the skull, and the spinal cord is contained within the vertebral cavity of the vertebral column. It is a bit of an oversimplification to say that the central nervous system is what is inside these two cavities and the peripheral nervous system is outside of them, but that is one way to start to think about it. In actuality, there are some elements of the peripheral nervous system that are within the cranial or vertebral cavities. The peripheral nervous system is so named because it is on the periphery—meaning beyond the brain and spinal cord. Depending on different aspects of the nervous system, the dividing line between central and peripheral is not necessarily universal.
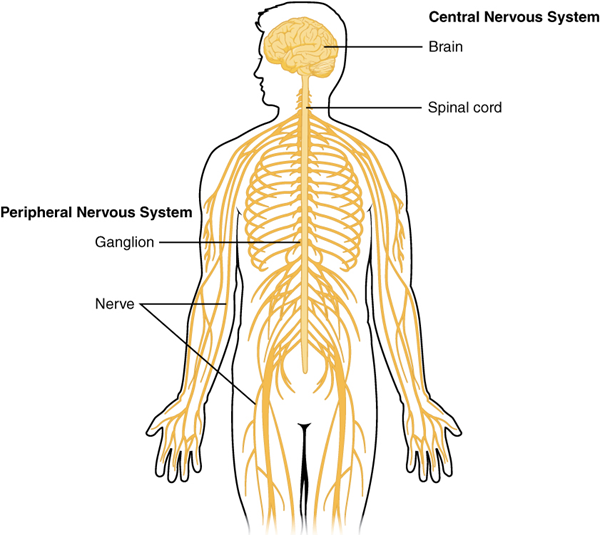
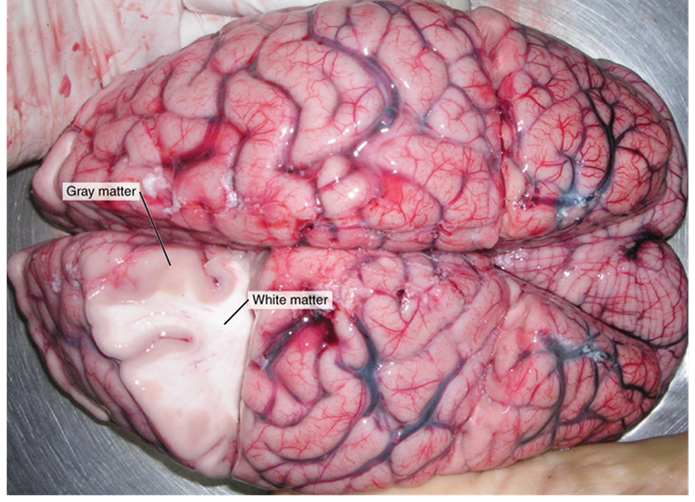
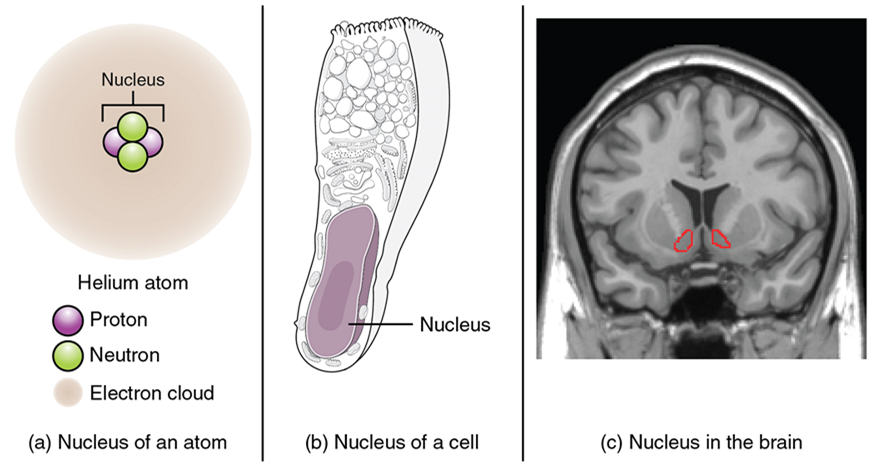
These two regions within nervous system structures are often referred to as gray matter (the regions with many cell bodies and dendrites) or white matter (the regions with many axons). The colors ascribed to these regions are what would be seen in “fresh,” or unstained, nervous tissue (Figure 15.3). Gray matter is not necessarily gray. It can be pinkish because of blood content, or even slightly tan, depending on how long the tissue has been preserved. But white matter is white because axons are insulated by a lipid-rich substance called myelin. Lipids can appear as white (“fatty”) material, much like the fat on a raw piece of chicken or beef. Gray matter may have that color ascribed to it because next to the white matter, it is just darker—hence, gray.The distinction between gray matter and white matter is most often applied to central nervous tissue, which has large regions that can be seen with the unaided eye. When looking at peripheral structures, often a microscope is used, and the tissue is stained with artificial colors. That is not to say that central nervous tissue cannot be stained and viewed under a microscope, but unstained tissue is most likely from the central nervous system—for example, a frontal section of the brain or cross section of the spinal cord.
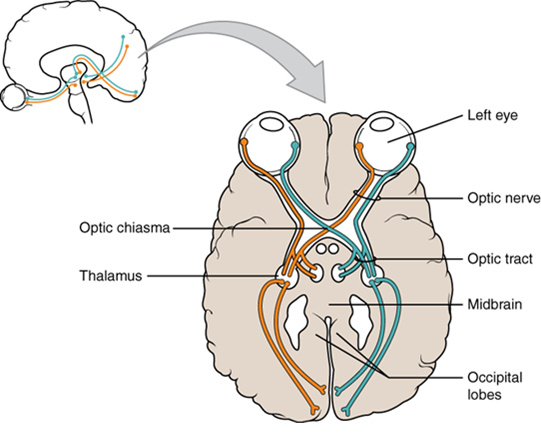
Regardless of the appearance of stained or unstained tissue, the cell bodies of neurons or axons can be located in discrete anatomical structures that require a name. Those names are specific to whether the structure is central or peripheral. A localized collection of neuron cell bodies in the central nervous system is referred to as a nucleus. In the peripheral nervous system, a cluster of neuron cell bodies is referred to as a ganglion. The term nucleus has a few different meanings within anatomy and physiology. It is the center of an atom, where protons and neutrons are found; it is the center of a cell, where genomic DNA is found; and it is a center of some function in the central nervous system (Figure 15.4). There is also a potentially confusing use of the word ganglion (plural = ganglia) that has a historical explanation. In the central nervous system, there is a group of nuclei that are connected together and were once called the basal ganglia before “ganglion” became accepted as a description for a peripheral structure. Some sources refer to this group of nuclei as the “basal nuclei” to avoid confusion.
CNS | PNS | |
---|---|---|
Group of neuron cell bodies (i.e., gray matter) | Nucleus | Ganglion |
Bundle of axons (i.e., white matter) | Tract | Nerve |
Terminology applied to bundles of axons also differs depending on location. A bundle of axons, or fibers, found in the central nervous system is called a tract, whereas the same thing in the peripheral nervous system would be called a nerve. There is an important point to make about these terms, which is that they can both be used to refer to the same bundle of axons. When those axons are located in the peripheral nervous system, the term is nerve, but if they are in the central nervous system, the term is tract. The most obvious example of this is the axons that project from the retina into the brain. Those axons are called the optic nerve, as they leave the eye, but when they are inside the cranium, they are referred to as the optic tract. There is a specific place where the name changes, which is the optic chiasm, but they are still the same axons (Figure 15.5). Table 15.1 helps clarify which of these terms apply to the central or peripheral nervous systems.
Functional Divisions
There are two ways to consider how the nervous system is divided functionally. First, the basic functions of the nervous system are sensation, integration, and response. Secondly, control of the body can be somatic or autonomic—divisions that are largely defined by the structures that are involved in the response (Figure 15.6). There is also a region of the peripheral nervous system that is called the enteric nervous system that is responsible for a specific set of the functions within the realm of autonomic control related to gastrointestinal functions.
Basic Functions: Sensation, Integration, and Response
The nervous system is involved in receiving information about the environment around us (sensation) and generating responses to that information (motor responses). The nervous system can be divided into regions that are responsible for sensation (sensory functions) and for the response (motor functions). But there is a third function that needs to be included. Sensory input needs to be integrated with other sensations, as well as with memories, emotional state, or learning (cognition). Some regions of the nervous system are termed integration or association areas. The process of integration combines sensory perceptions and higher cognitive functions such as memories, learning, and emotion to produce a response.
The first major function of the nervous system is sensation—receiving information about the environment to gain input about what is happening outside the body (or sometimes, within the body). The sensory functions of the nervous system register the presence of a particular event in the external or internal environment, known as a stimulus. The senses we think of most are the “big five”: taste, smell, touch, sight, and hearing. The stimuli for taste and smell are both chemical substances (molecules, compounds, ions, etc.), touch is physical or mechanical stimuli that interact with the skin, sight is light stimuli, and hearing is the perception of sound, which is a physical stimulus similar to some aspects of touch. There are actually more senses than just those, but that list represents the major senses. These five are all senses that receive stimuli from the outside world, and of which there is conscious perception. Additional sensory stimuli might be from the internal environment (inside the body), such as the stretch of an organ wall or the concentration of certain ions in the blood.
Stimuli that are received by sensory structures are communicated to the nervous system, where that information is processed. This is called integration. Stimuli are compared with, or integrated with, other stimuli, memories of previous stimuli, or the state of a person at a particular time. This leads to the specific response that will be generated. Seeing a baseball pitched to a batter will not automatically cause the batter to swing. The trajectory of the ball and its speed will need to be considered. Maybe the count is three balls and no strikes, and the batter wants to let this pitch go by in the hope of getting a walk to first base. Or maybe the batter’s team is so far ahead, it would be fun to just swing away.
The nervous system produces a response on the basis of the stimuli perceived by sensory structures. An obvious response would be the movement of muscles, such as withdrawing a hand from a hot stove, but there are broader uses of the term. The nervous system can cause the contraction of all three types of muscle tissue. For example, skeletal muscle contracts to move the skeleton, cardiac muscle is stimulated as the heart rate increases during exercise, and smooth muscle contracts as the digestive system moves food along the digestive tract. Responses also include the neural control of glands in the body as well, such as the production and secretion of sweat by the eccrine and apocrine sweat glands found in the skin to lower body temperature.
Responses can be divided into those that are voluntary or conscious (contraction of skeletal muscle) and those that are involuntary (contraction of smooth muscle, regulation of cardiac muscle, activation of glands). Voluntary responses are governed by the somatic nervous system, and involuntary responses are governed by the autonomic nervous system, which are discussed in the next section.
Somatic, Autonomic and Enteric Nervous Systems
The nervous system can be divided into two parts, mostly on the basis of a functional difference in responses. The somatic nervous system (SNS) is responsible for conscious perception and voluntary motor responses. Voluntary motor response means the contraction of skeletal muscle, but those contractions are not always voluntary in the sense that you have to want to perform them. Some somatic motor responses are reflexes and often happen without a conscious decision to perform them. If your friend jumps out from behind a corner and yells “Boo!” you will be startled, and you might scream or leap back. You didn’t decide to do that, and you may not have wanted to give your friend a reason to laugh at your expense, but it is a reflex involving skeletal muscle contractions. Other motor responses become automatic (in other words, unconscious) as a person learns motor skills (referred to as “habit learning” or “procedural memory”).
The autonomic nervous system (ANS) is responsible for the involuntary control of the body, usually for the sake of homeostasis (regulation of the internal environment). Sensory input for autonomic functions can be from sensory structures tuned to external or internal environmental stimuli. The motor output extends to smooth and cardiac muscle as well as glandular tissue. The role of the autonomic system is to regulate the organ systems of the body, which usually means to control homeostasis. Sweat glands, for example, are controlled by the autonomic system. When you are hot, sweating helps cool your body down. That is a homeostatic mechanism. But when you are nervous, you might start sweating also. That is not homeostatic; it is the physiological response to an emotional state.
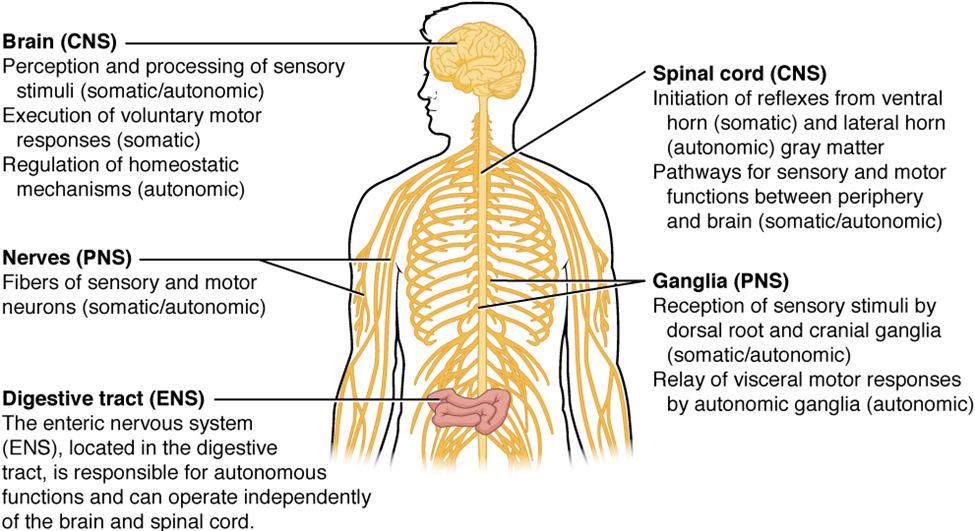
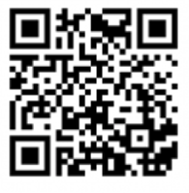
Test Your Knowledge
I. Describe the organization of the nervous system and explain the functions of its principal components.
- Draw a flow chart demonstrating the relationships between, and stating the main function of each of the following components of the nervous system:
-
-
- Central nervous system
- Peripheral nervous system
- Sensory neurons
- Motor neurons
- Somatic nervous system
- Autonomic nervous system
- Sympathetic nervous system
- Parasympathetic nervous system
-
Part 2: Nervous Tissue
Nervous tissue is composed of two types of cells, neurons, and glial cells. Neurons are the primary type of cell that most anyone associates with the nervous system. They are responsible for the computation and communication that the nervous system provides. They are electrically active and release chemical signals to target cells. Glial cells, or glia, are known to play a supporting role for nervous tissue. Ongoing research pursues an expanded role that glial cells might play in signaling, but neurons are still considered the basis of this function. Neurons are important, but without glial support, they would not be able to perform their function.
Neurons
Neurons are the cells considered to be the basis of nervous tissue. They are responsible for the electrical signals that communicate information about sensations and that produce movements in response to those stimuli, along with inducing thought processes within the brain. An important part of the function of neurons is in their structure, or shape. The three-dimensional shape of these cells makes the immense numbers of connections within the nervous system possible.
Parts of a Neuron
As you learned in the first section, the main part of a neuron is the cell body, which is also known as the soma (soma = “body”). The cell body contains the nucleus and most of the major organelles. But what makes neurons special is that they have many extensions of their cell membranes, which are generally referred to as processes. Neurons are usually described as having one, and only one, axon—a fiber that emerges from the cell body and projects to target cells (Figure 15.6). That single axon can branch repeatedly to communicate with many target cells. It is the axon that propagates the nerve impulse, which is communicated to one or more cells. The other processes of the neuron are dendrites (Figure 15.6), which receive information from other neurons at specialized areas of contact called synapses. The dendrites are usually highly branched processes, providing locations for other neurons to communicate with the cell body. Information flows through a neuron from the dendrites, across the cell body, and down the axon. This gives the neuron a polarity—meaning that information flows in this one direction.
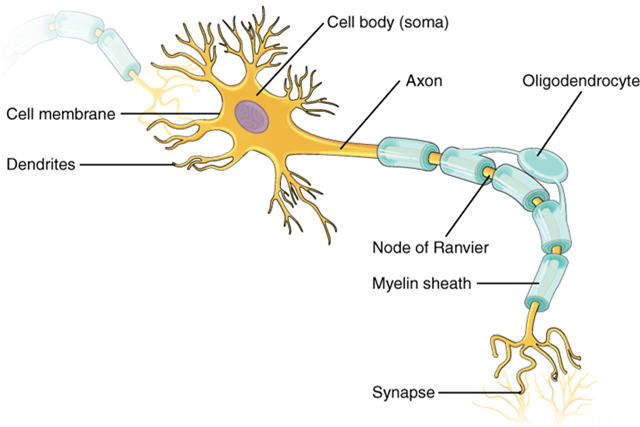
Types of Neurons
There are many neurons in the nervous system—a number in the trillions. And there are many different types of neurons. They can be classified by many different criteria. The first way to classify them is by the number attached to the cell body. Using the standard model of neurons, one of these processes is the axon, and the rest are dendrites. Because information flows through the neuron from dendrites or cell bodies toward the axon, these names are based on the neuron’s (Figure 15.7).
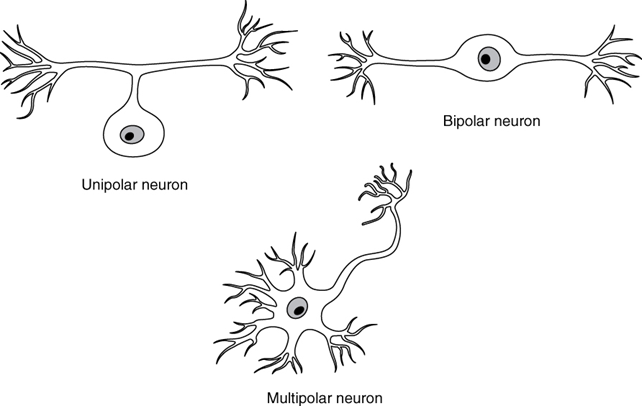
Neurons can also be classified on the basis of where they are found, who found them, what they do, or even what chemicals they use to communicate with each other. Some neurons referred to in this section on the nervous system are named on the basis of those sorts of classifications (Figure 15.8). For example, a multipolar neuron that has a very important role to play in a part of the brain called the cerebellum is known as a Purkinje (commonly pronounced per-KIN-gee) cell. It is named after the anatomist who discovered it (Jan Evangelista Purkinje, 1787–1869).
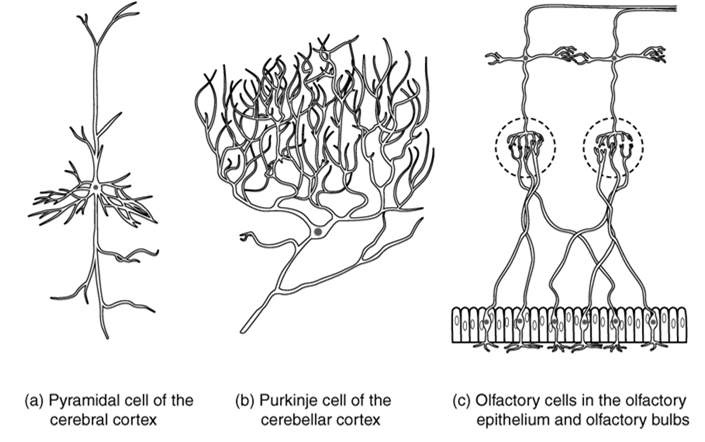
Glial Cells
Glial cells, or neuroglia, or simply glia, are the other type of cell found in nervous tissue. They are considered to be supporting cells, and many functions are directed at helping neurons complete their function for communication. The name glia comes from the Greek word that means “glue” and was coined by the German pathologist Rudolph Virchow, who wrote in 1856, “This connective substance, which is in the brain, the spinal cord, and the special sense nerves, is a kind of glue (neuroglia) in which the nervous elements are planted.” Today, research into nervous tissue has shown that there are many deeper roles that these cells play. And research may find much more about them in the future.
CNS glia | PNS glia | Basic function |
---|---|---|
Astrocyte | Satellite cell | Support |
Oligodendrocyte | Schwann cell | Insulation, myelination |
Microglia | – | Immune surveillance, phagocytosis |
Ependymal cell | – | Creating cerebrospinal fluid |
There are six types of glial cells (Table 2). Four of them are found in the central nervous system (Figure 15.9), and two are found in the peripheral nervous system (Figure 15.10). For reference, Table 2 outlines some common characteristics and functions of the various glial cell types.
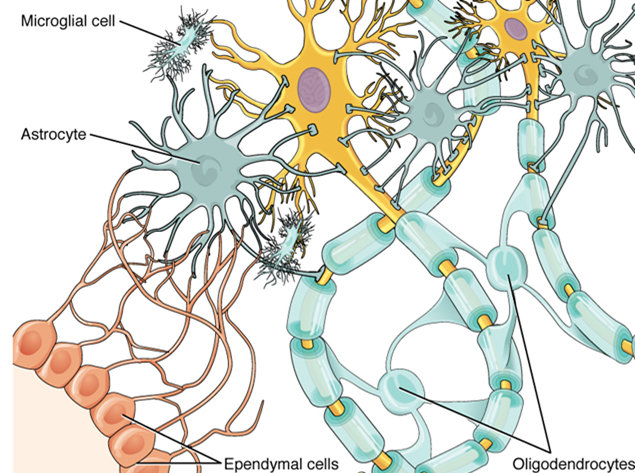
Myelin
The insulation for axons in the nervous system is provided by glial cells: oligodendrocytes in the central nervous system and Schwann cells in the peripheral nervous system. Whereas the manner in which either cell is associated with the axon segments, or segments, that it insulates is different, the means of myelinating an axon segment is mostly the same in the two situations. Myelin is a lipid-rich sheath that surrounds the axon and by doing so creates a myelin sheath that facilitates the transmission of electrical signals along the axon. The lipids are essentially the phospholipids of the glial cell membrane. Myelin, however, is more than just the membrane of the glial cell. It also includes important proteins that are integral to that membrane. Some of the proteins help to hold the layers of the glial cell membrane closely together.
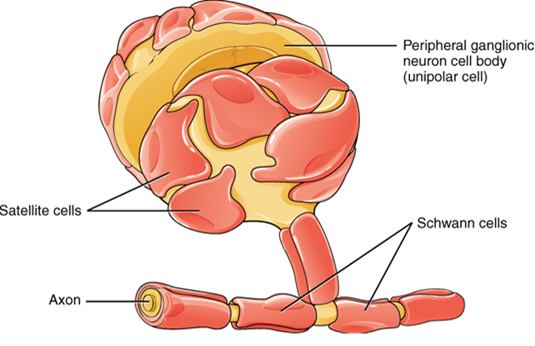
Test Your Knowledge
II. Describe the structure of the following: neuron, glia, ganglion, nerve, gray matter, tract, white matter, sensory neuron, motor neuron.
- Name the parts of a typical neuron and describe their functions.
- Compare and contrast the location, structure, and function of:
-
- Neurons and glia
- Nerves and tracts
- White matter and nerves
- White matter and gray matter
- Nerves and ganglia
- Ganglia and gray matter
- Sensory and motor neurons
Part 3: The Central Nervous System
The brain and the spinal cord are the central nervous system, and they represent the main organs of the nervous system. The spinal cord is a single structure, whereas the adult brain is described in terms of four major regions: the cerebrum, the diencephalon, the brain stem, and the cerebellum. A person’s conscious experiences are based on neural activity in the brain. The regulation of homeostasis is governed by a specialized region in the brain. The coordination of reflexes depends on the integration of sensory and motor pathways in the spinal cord.
The Cerebrum
The iconic gray mantle of the human brain, which appears to make up most of the mass of the brain, is the cerebrum with two distinct halves, a right and left cerebral hemisphere (Figure 15.11). Many of the higher neurological functions, such as memory, emotion, and consciousness, are the result of cerebral function. The cerebrum comprises a continuous, wrinkled, and thin layer of gray matter that wraps around both hemispheres, the cerebral cortex, and several deep nuclei. A (plural = gyri) is the ridge of one of those wrinkles, and a (plural = sulci) is the groove between two gyri. The pattern of these folds of tissue indicates specific regions of the cerebral cortex (Figure 15.12).
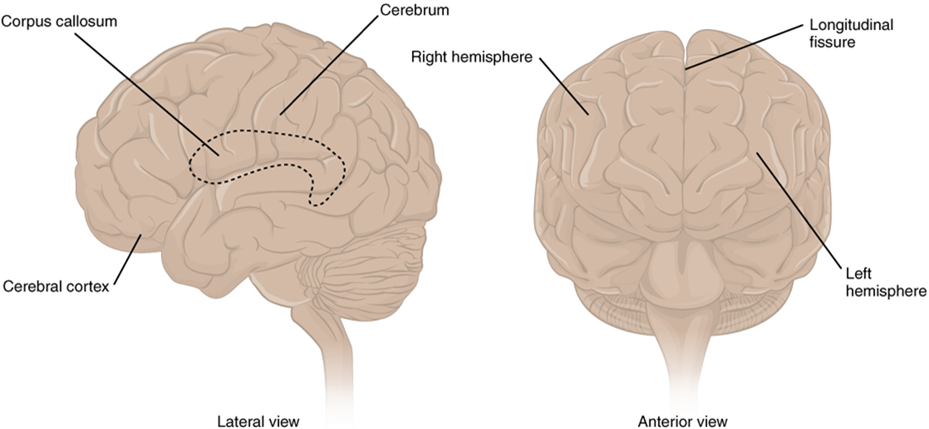
Different regions of the cerebral cortex can be associated with particular functions, a concept known as localization of function. In the early 1900s, a German neuroscientist named Korbinian Brodmann performed an extensive study of the microscopic anatomy (cytoarchitecture) of the cerebral cortex and divided the cortex into 52 separate regions on the basis of the histology of the cortex. His work resulted in a system of classification known as Brodmann's areas, which is still used today to describe the anatomical distinctions within the cortex. The results from Brodmann’s work on the anatomy align very well with the functional differences within the cortex. For example, Areas 17 and 18 in the occipital lobe are responsible for primary visual perception. That visual information is complex, so it is processed in the temporal and parietal lobes as well.
Beneath the cerebral cortex are sets of nuclei known as basal nuclei that augment cortical processes (Figure 15.13). Some of the basal nuclei in the forebrain, for example, serve as the primary location for acetylcholine production, which modulates the overall activity of the cortex, possibly leading to greater attention to sensory stimuli. Alzheimer’s disease is associated with a loss of neurons in the cholinergic basal forebrain nuclei. Some other basal nuclei control the initiation of movement. For example, while a student is sitting in a classroom listening to a lecture, the basal nuclei will keep an urge to jump up and scream from actually happening. (The basal nuclei are also referred to as the basal ganglia, although that is potentially confusing because the term ganglia is typically used for peripheral structures.)
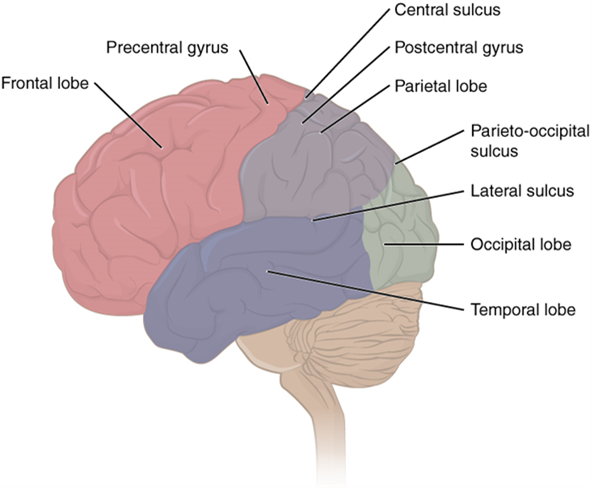
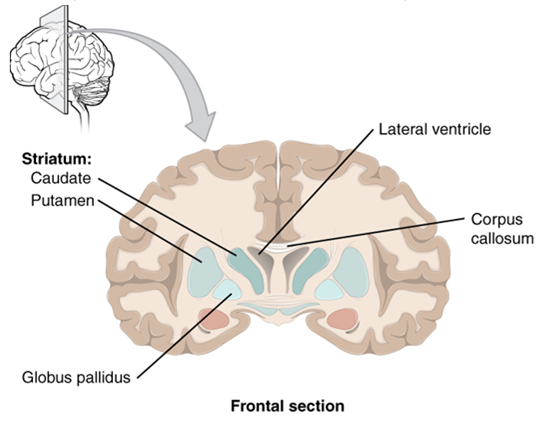
The Diencephalon
The word diencephalon translates to “through brain.” It is the connection between the cerebrum and the rest of the nervous system, with one exception. The rest of the brain, the spinal cord, and the peripheral nervous system all send information to the cerebrum through the diencephalon. Output from the cerebrum passes through the diencephalon. The single exception is the system associated with olfaction, or the sense of smell, which connects directly with the cerebrum.
The diencephalon is deep beneath the cerebrum and constitutes the walls of the third ventricle. The diencephalon can be described as any region of the brain with “thalamus” in its name. The two major regions of the diencephalon are the thalamus itself and the hypothalamus (Figure 15.14). There are other structures, such as the epithalamus, which contains the pineal gland, and the subthalamus, which includes the subthalamic nucleus, one of the basal nuclei.
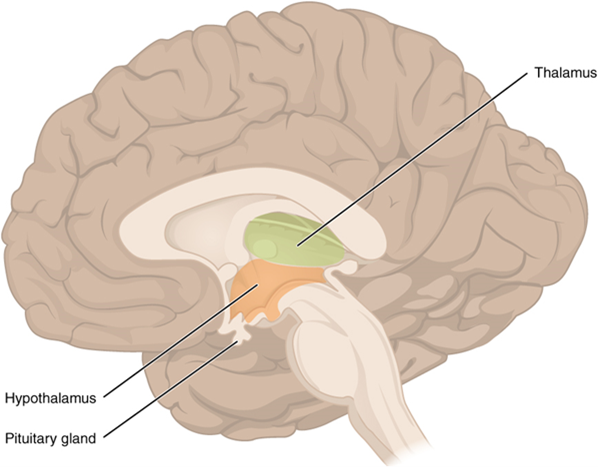
Thalamus
The thalamus is a collection of nuclei that relay information between the cerebral cortex and the periphery, spinal cord, or brain stem. All sensory information, except for the sense of smell, passes through the thalamus before processing by the cortex. Axons from the peripheral sensory organs, or intermediate nuclei, synapse in the thalamus, and thalamic neurons project directly to the cerebrum. It is a requisite synapse in any sensory pathway, except for olfaction. The thalamus does not just pass the information on, it also processes that information. For example, the portion of the thalamus that receives visual information will influence what visual stimuli are important, or what receives attention. The cerebrum also sends information down to the thalamus, which usually communicates motor commands.
Hypothalamus
Inferior and slightly anterior to the thalamus is the hypothalamus, the other major region of the diencephalon. The hypothalamus is a collection of nuclei that are largely involved in regulating homeostasis. The hypothalamus is the executive region in charge of the autonomic nervous system and the endocrine system through its regulation of the anterior. Other parts of the hypothalamus are involved in memory and emotion as part of the limbic system.
The Brain Stem
The midbrain and hindbrain (composed of the pons and the medulla) are collectively referred to as the brain stem (Figure 15.15). The structure emerges from the ventral surface of the forebrain as a tapering cone that connects the brain to the spinal cord. Attached to the brain stem, but considered a separate region of the adult brain, is the cerebellum. The midbrain coordinates sensory representations of the visual, auditory, and somatosensory perceptual spaces. The pons is the main connection with the cerebellum. The pons and the medulla regulate several crucial functions, including the cardiovascular and respiratory systems.
The cranial nerves connect through the brain stem and provide the brain with the sensory input and motor output associated with the head and neck, including most of the special senses. The major ascending and descending pathways between the spinal cord and brain, specifically the cerebrum, pass through the brain stem.
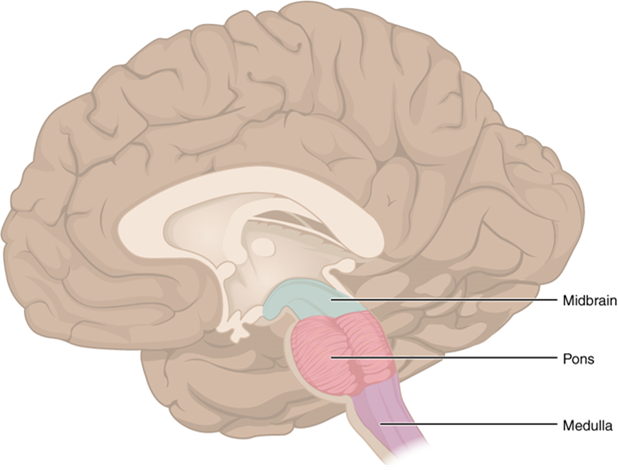
Midbrain
One of the original regions of the embryonic brain, the midbrain is a small region between the thalamus and pons. The cerebral aqueduct passes through the center of the midbrain, such that these regions are the roof and floor of that canal.
The midbrain includes four bumps known as the colliculi (singular = colliculus), which means “little hill” in Latin. The inferior colliculus is the inferior pair of these enlargements and is part of the auditory brain stem pathway. Neurons of the inferior colliculus project to the thalamus, which then sends auditory information to the cerebrum for the conscious perception of sound. The superior colliculus is the superior pair and combines sensory information about visual space, auditory space, and somatosensory space. Activity in the superior colliculus is related to orienting the eyes to a sound or touch stimulus. If you are walking along the sidewalk on campus and you hear chirping, the superior colliculus coordinates that information with your awareness of the visual location of the tree right above you. That is the correlation of auditory and visual maps. If you suddenly feel something wet fall on your head, your superior colliculus integrates that with the auditory and visual maps, and you know that the chirping bird just relieved itself on you. You want to look up to see the culprit but do not.
Pons
The word pons comes from the Latin word for bridge. It is visible on the anterior surface of the brain stem as the thick bundle of white matter attached to the cerebellum. The pons is the main connection between the cerebellum and the brain stem.
Medulla
The gray matter of the midbrain and pons continues into the medulla, also known as medulla oblongata. This diffuse region of gray matter throughout the brain stem, known as the reticular formation, is related to sleep and wakefulness, general brain activity, and attention. The medulla contains autonomic nuclei with motor neurons that control the rate and force of heart contraction, the diameter of blood vessels, and the rate and depth of breathing, among other essential physiological processes.
The Cerebellum
The cerebellum, as the name suggests, is the “little brain.” It is covered in gyri and sulci like the cerebrum and looks like a miniature version of that part of the brain (Figure 15.16). The cerebellum integrates motor commands from the cerebral cortex with sensory feedback from the periphery, allowing for the coordination and precise execution of motor activities, such as walking, cycling, writing, or playing a musical instrument.
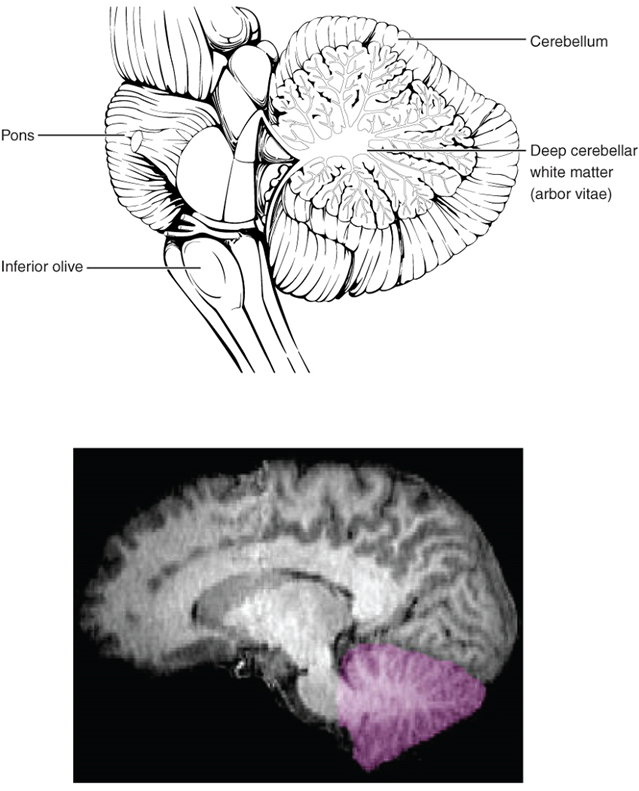
The Spinal Cord
Whereas the brain develops out of expansions of the neural tube into primary and then secondary vesicles, the spinal cord maintains the tube structure and is only specialized into certain regions.
The length of the spinal cord is divided into regions that correspond to the regions of the vertebral column. The name of a spinal cord region corresponds to the level at which spinal nerves pass through the intervertebral foramina. Immediately adjacent to the brain stem is the cervical region, followed by the thoracic, then the lumbar, and finally the sacral region (Figure 15.17).
Gray Horns
In cross section, the gray matter of the spinal cord has the appearance of an ink-blot test, with the spread of the gray matter on one side replicated on the other—a shape reminiscent of a bulbous capital “H.” As shown in Figure 19, the gray matter is subdivided into regions that are referred to as horns.
The posterior horn is responsible for sensory processing. The anterior horn sends out motor signals to the skeletal muscles. The lateral horn, which is only found in the thoracic, upper lumbar, and sacral regions, is the central component of the sympathetic division of the autonomic nervous system.
Some of the largest neurons of the spinal cord are the multipolar motor neurons in the anterior horn. The fibers that cause the contraction of skeletal muscles are the axons of these neurons. The motor neuron that causes contraction of the big toe, for example, is located in the sacral spinal cord. The axon that has to reach all the way to the belly of that muscle may be a meter in length. The neuronal cell body that maintains that long fiber must be quite large, possibly several hundred micrometers in diameter, making it one of the largest cells in the body.
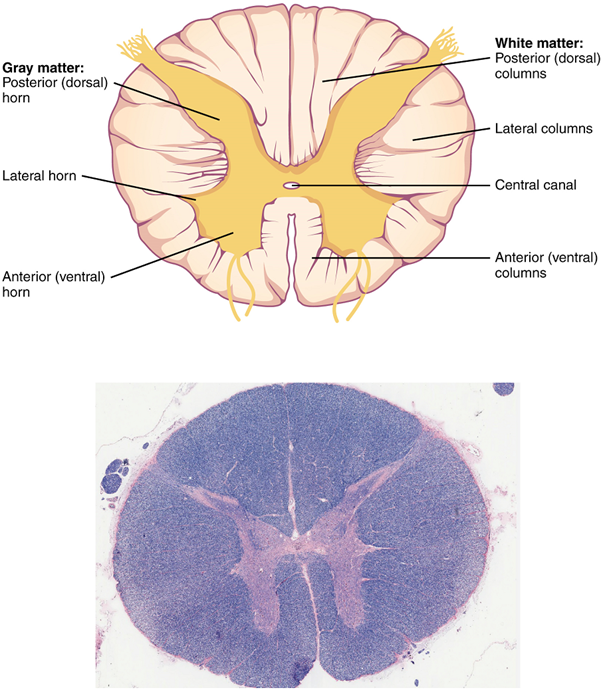
White Columns
Just as the gray matter is separated into horns, the white matter of the spinal cord is separated into columns. Ascending tracts of nervous system fibers in these columns carry sensory information up to the brain, whereas descending tracts carry motor commands from the brain.
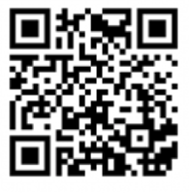
The Meninges
The outer surface of the central nervous system is covered by a series of membranes composed of connective tissue called the meninges, which protect the brain. The dura mater is a thick fibrous layer and a strong protective sheath over the entire brain and spinal cord. It is anchored to the inner surface of the cranium and vertebral cavity. The arachnoid mater is a membrane of thin fibrous tissue that forms a loose sac around the central nervous system. Beneath the arachnoid is a thin, filamentous mesh called the arachnoid trabeculae, which looks like a spider web, giving this layer its name. Directly adjacent to the surface of the central nervous system is the pia mater, a thin fibrous membrane that follows the convolutions of gyri and sulci in the cerebral cortex and fits into other grooves and indentations (Figure 15.18).
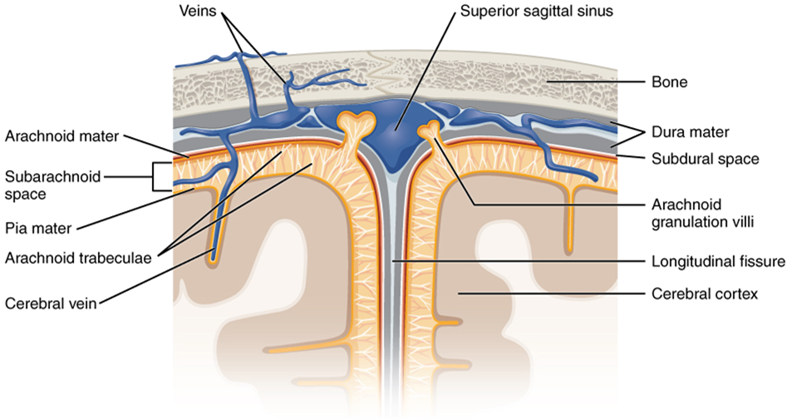
The Ventricular System and Cerebrospinal Fluid Circulation
Cerebrospinal fluid (CSF) circulates throughout and around the central nervous system. Cerebrospinal fluid is produced in special structures to perfuse through the nervous tissue of the central nervous system and is continuous with the interstitial fluid. Specifically, cerebrospinal fluid circulates to remove metabolic wastes from the interstitial fluids of nervous tissues and return them to the bloodstream. The ventricles are the open spaces within the brain where cerebrospinal fluid circulates. In some of these spaces, cerebrospinal fluid is produced by filtering of the blood that is performed by a specialized membrane known as a choroid plexus. The cerebrospinal fluid circulates through all of the ventricles to eventually emerge into the subarachnoid space, where it is reabsorbed into the blood.
There are four ventricles within the brain, all of which developed from the original hollow space within the neural tube, the central canal. The first two are named the lateral ventricles and are deep within the cerebrum. These ventricles are connected to the third ventricle by two openings called the interventricular foramina. The third ventricle is the space between the left and right sides of the diencephalon, which opens into the cerebral aqueduct that passes through the midbrain. The aqueduct opens into the fourth ventricle, which is the space between the cerebellum and the pons and upper medulla (Figure 15.19).
The ventricular system opens up to the subarachnoid space from the fourth ventricle. The single median aperture and the pair of lateral apertures connect to the subarachnoid space so that cerebrospinal fluid can flow through the ventricles and around the outside of the central nervous system. Cerebrospinal fluid is produced within the ventricles by a type of specialized membrane called a choroid plexus. Ependymal cells (a type of glial cell; see Figure 15.11) surround blood capillaries and filter the blood to make cerebrospinal fluid. The fluid is a clear solution with a limited amount of the constituents of blood. It is essentially water, small molecules, and electrolytes. Oxygen and carbon dioxide are dissolved into the cerebrospinal fluid, as they are in blood, and can diffuse between the fluid and the nervous tissue.
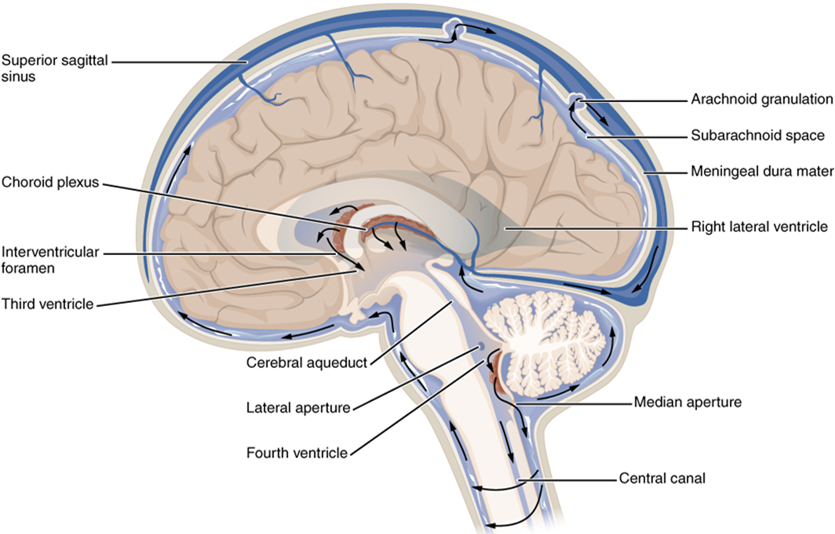
Cerebrospinal Fluid Circulation
The choroid plexuses are found in all four ventricles. Observed in dissection, they appear as soft, fuzzy structures that may still be pink, depending on how well the circulatory system is cleared in preparation of the tissue. The CSF is produced from components extracted from the blood, so its flow out of the ventricles is tied to the pulse of cardiovascular circulation.
From the lateral ventricles, the CSF flows into the third ventricle, where more CSF is produced, and then through the cerebral aqueduct into the fourth ventricle where even more CSF is produced. A very small amount of CSF is filtered at any one of the plexuses, for a total of about 500 milliliters daily, but it is continuously made and pulses through the ventricular system, keeping the fluid moving. From the fourth ventricle, CSF can continue down the central canal of the spinal cord, but this is essentially a cul-de-sac, so more of the fluid leaves the ventricular system and moves into the subarachnoid space through the median and lateral apertures.
Within the subarachnoid space, the cerebrospinal fluid flows around all of the central nervous system, providing two important functions. As with elsewhere in its circulation, the cerebrospinal fluid picks up metabolic wastes from the nervous tissue and moves it out of the central nervous system. It also acts as a liquid cushion for the brain and spinal cord. By surrounding the entire system in the subarachnoid space, it provides a thin buffer around the organs within the strong, protective dura mater. The arachnoid granulations are outpocketings of the arachnoid membrane into the dural sinuses so that cerebrospinal fluid can be reabsorbed into the blood, along with the metabolic wastes. From the dural sinuses, blood drains out of the head and neck through the jugular veins, along with the rest of the circulation for blood, to be re-oxygenated by the lungs and wastes to be filtered out by the kidneys (Table 15.3).
Lateral ventricles | Third ventricle | Cerebral aqueduct | Fourth ventricle | Central canal | Subarachnoid space | |
---|---|---|---|---|---|---|
Location | Cerebrum | Diencephalon | Midbrain | Between pons/upper medulla oblongata and cerebellum | Spinal cord | External to entire central nervous system |
Blood vessel structure | Choroid plexus | Choroid plexus | None | Choroid plexus | None | Arachnoid granulations |
Test Your Knowledge
III. Name, locate and describe the functions of the main areas of the human brain.
- Describe the general anatomy of the brain, including the location of the lobes.
- Where in the brain would you find the cell bodies of neurons? Where would you find their axons? Describe how you can tell just by looking at a (cut) brain with the naked eye.
- Describe the location and function of each of the following areas of the human brain:
- Cerebrum
- Diencephalon
- Thalamus
- Hypothalamus
- Brain stem
- Midbrain
- Pons
- Medulla oblongata
- Cerebellum
- What are the names of the three meninges, and where are they located?
- What are the names of the four ventricles, and where are they located?
- Describe the path taken by cerebrospinal fluid through the brain.
IV. Describe the structure and explain the functions of the spinal cord.
- Where in the spinal cord would you find the cell bodies of neurons? Where would you find their axons? Describe how you can tell just by looking at a (cut) spinal cord with the naked eye.
- What are some of the functions of the spinal cord?
Part 4: The Peripheral Nervous System
The peripheral nervous system is not as contained as the central nervous system because it is defined as everything that is not the central nervous system. Some peripheral structures are incorporated into the other organs of the body. In describing the anatomy of the peripheral nervous system, it is necessary to describe the common structures, the nerves and the ganglia, as they are found in various parts of the body. Many of the neural structures that are incorporated into other organs are features of the digestive system; these structures are known as the enteric nervous system and are a special subset of the peripheral nervous system.
Ganglia
A ganglion is a group of neuron cell bodies in the periphery. Ganglia can be categorized, for the most part, as either sensory ganglia or autonomic ganglia, referring to their primary functions. The most common type of sensory ganglion is a dorsal root ganglion. These ganglia are the cell bodies of neurons with axons that are sensory endings in the periphery, such as in the skin, and that extend into the central nervous system through the dorsal nerve root (Figure 15.20).
The other major category of ganglia, those of the autonomic nervous system, will be examined later in this chapter.
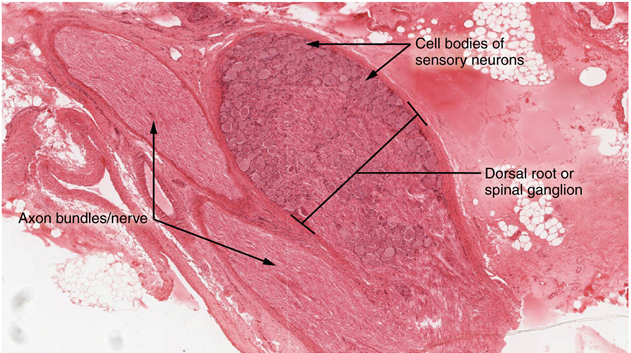
Nerves
Bundles of axons in the peripheral nervous system are referred to as nerves. These structures in the periphery are different than their central counterpart, called a tract. Nerves are composed of more than just nervous tissue. They have connective tissues invested in their structure, as well as blood vessels supplying the tissues with nourishment. Nerves are associated with the region of the central nervous system to which they are connected, either as cranial nerves (12 pairs) connected to the brain or spinal nerves (31 pairs) connected to the spinal cord.
The cranial nerves are primarily responsible for the sensory and motor functions of the head and neck, although one of these nerves, the vagus, targets organs in the thoracic and abdominal cavities as part of the parasympathetic nervous system. They can be classified as sensory nerves, motor nerves, or a combination of both, meaning that the axons in these nerves originate out of sensory ganglia external to the cranium or motor nuclei within the brain stem.
All of the spinal nerves are combined sensory and motor axons that separate into two nerve roots. The sensory axons enter the spinal cord as the dorsal nerve root. The motor fibers, both somatic and autonomic, emerge as the ventral nerve root. The dorsal root ganglion for each nerve is an enlargement of the spinal nerve.
The Somatic Nervous System
The somatic nervous system is traditionally considered a division within the peripheral nervous system. However, this misses an important point: somatic refers to a functional division, whereas peripheral refers to an anatomic division. The somatic nervous system is responsible for our conscious perception of the environment and for our voluntary responses to that perception by means of skeletal muscles. Peripheral sensory neurons receive input from environmental stimuli, but the neurons that produce motor responses originate in the central nervous system. The distinction between the structures of the peripheral and central nervous systems and the functions of the somatic and autonomic systems can most easily be demonstrated through a simple reflex, an automatic response that the nervous system produces in response to specific stimuli. The neurons and neural pathways responsible for a reflex action constitute the reflex arc. One of the simplest reflex acts is the stretch reflex, by which the nervous system responds to the stretching of a muscle (the stimulus) with the contraction of that same muscle (the response). This response protects the muscle from over-stretching, but more importantly, it has a crucial role in maintaining posture and balance. The patellar reflex (or knee-jerk reflex) is an example of stretch reflex, and it occurs through the following steps (Figure 15.21):
- Tapping of the patellar tendon with a hammer causes the stretching of muscle fibers in the quadriceps muscle, which stimulates sensory neurons innervating those fibers.
- In the sensory neuron, a nerve impulse (action potential) is generated, which propagates along the sensory nerve fiber from the muscle, through the dorsal root ganglion, to the spinal cord.
- The sensory neuron stimulates a motor neuron in the ventral horn of the spinal cord.
- That motor neuron sends a nerve impulse (action potential) along its axon.
- This impulse reaches the quadriceps muscle, causing its contraction and the extension of the leg (a kick).
The sensory neuron can also activate an interneuron (e.g., Figure 15.21), which inhibits the motor neuron responsible for the contraction of the antagonistic muscle to quadriceps (i.e., hamstring).
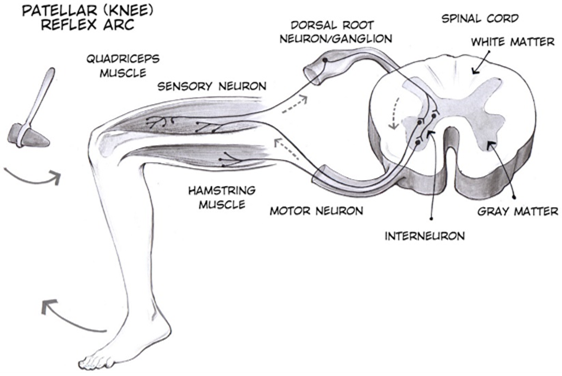
Another example of a simple spinal reflex is the withdrawal reflex, which occurs, for example, when you touch a hot stove and pull your hand away. This reflex occurs through a similar sequence of steps:
- Sensory receptors in the skin sense extreme temperature and the early signs of tissue damage.
- In a sensory neuron, a nerve impulse (action potential) is generated, which propagates along the sensory nerve fiber from the skin, through the dorsal root ganglion, to the spinal cord.
- The sensory neuron stimulates a motor neuron in the ventral horn motor of the spinal cord.
- That motor neuron sends a nerve impulse (action potential) along its axon.
- This impulse reaches the biceps brachii, causing contraction of the muscle and flexion of the forearm at the elbow to withdraw the hand from the hot stove.
The basic withdrawal reflex includes sensory input (the painful stimulus), central processing (the synapse in the spinal cord), and motor output (activation of a ventral motor neuron that causes contraction of the biceps brachii). As seen for the patellar reflex, the withdrawal reflex can also include inhibition of the antagonistic muscle (triceps brachii in our example). Another possible motor output of the withdrawal reflex is cross extension: counterbalancing movement on the other side of the body by stimulation of the extensor muscles in the contralateral limb.
The somatic nervous system also controls voluntary movement and more complex motor functions. For example, reading of this text starts with visual sensory input to the retina, which then projects to the thalamus, and on to the cerebral cortex. A sequence of regions of the cerebral cortex process the visual information, starting in the primary visual cortex of the occipital lobe and resulting in the conscious perception of these letters. Subsequent cognitive processing results in understanding of the content. As you continue reading, regions of the cerebral cortex in the frontal lobe plan how to move the eyes to follow the lines of text. The output from the cortex causes activity in motor neurons in the brain stem that cause movement of the extraocular muscles through the third, fourth, and sixth cranial nerves. This example also includes sensory input (the retinal projection to the thalamus), central processing (the thalamus and subsequent cortical activity), and motor output (activation of neurons in the brain stem that lead to coordinated contraction of extraocular muscles).
The Autonomic Nervous System
The autonomic nervous system is often associated with the “fight-or-flight response,” which refers to the preparation of the body to either run away from a threat or to stand and fight in the face of that threat. To suggest what this means, consider the (very unlikely) situation of seeing a lioness hunting out on the savannah. Though this is not a common threat that humans deal with in the modern world, it represents the type of environment in which the human species adapted and thrived. The spread of humans around the world to the present state of the modern age occurred much more quickly than any species would adapt to environmental pressures such as predators. However, the reactions humans have in the modern world are based on these prehistoric situations. If your boss is walking down the hallway on Friday afternoon looking for “volunteers” to come in on the weekend, your response is the same as the prehistoric human seeing the lioness running across the savannah: fight or flight.
Most likely, your response to your boss—not to mention the lioness—would be flight. Run away! The autonomic system is responsible for the physiological response to make that possible, and hopefully successful. Adrenaline starts to flood your circulatory system. Your heart rate increases. Sweat glands become active. The bronchi of the lungs dilate to allow more air exchange. Pupils dilate to increase visual information. Blood pressure increases in general, and blood vessels dilate in skeletal muscles. Time to run. Similar physiological responses would occur in preparation for fighting off the threat.
This response should sound a bit familiar. The autonomic nervous system is tied into emotional responses as well, and the fight-or-flight response probably sounds like a panic attack. In the modern world, these sorts of reactions are associated with anxiety as much as with response to a threat. It is engrained in the nervous system to respond this way. In fact, the adaptations of the autonomic nervous system probably pre-date the human species and are likely to be common to all mammals. That lioness might herself be threatened in some other situation
However, the autonomic nervous system is not just about responding to threats. Besides the fight-or-flight response, there are the responses referred to as “rest and digest.” If that lioness is successful in her hunting, then she is going to rest from the exertion. Her heart rate will slow. Breathing will return to normal. The digestive system has a big job to do. Much of the function of the autonomic system is based on the connections within an autonomic, or visceral, reflex.
As we have seen, the nervous system can be divided into two functional parts: the somatic nervous system and the autonomic nervous system. The major differences between the two systems are evident in the responses that each produces. The somatic nervous system causes the contraction of skeletal muscles. The autonomic nervous system controls cardiac and smooth muscle, as well as glandular tissue. The somatic nervous system is associated with voluntary responses (though many can happen without conscious awareness, like breathing), and the autonomic nervous system is associated with involuntary responses, such as those related to homeostasis.
The autonomic nervous system regulates many of the internal organs through a balance of two aspects, or divisions. In addition to the endocrine system, the autonomic nervous system is instrumental in homeostatic mechanisms in the body. The two divisions of the autonomic nervous system are the sympathetic division and the parasympathetic division. The sympathetic system is associated with the fight-or-flight response, and parasympathetic activity is referred to by the epithet of rest and digest. At each target effector, dual innervation determines activity. For example, the heart receives connections from both the sympathetic and parasympathetic divisions. One causes heart rate to increase, whereas the other causes heart rate to decrease.
Sympathetic Division of the Autonomic Nervous System
To respond to a threat—to fight or to run away—the sympathetic system causes divergent effects, as many different effector organs are activated together for a common purpose. More oxygen needs to be inhaled and delivered to skeletal muscle. The respiratory, cardiovascular, and musculoskeletal systems are all activated together. Additionally, sweating keeps the excess heat that comes from muscle contraction from causing the body to overheat. The digestive system shuts down so that blood is not absorbing nutrients when it should be delivering oxygen to skeletal muscles. To coordinate all these responses, the connections in the sympathetic system diverge from a limited region of the central nervous system to a wide array of ganglia that project to the many effector organs simultaneously. The complex set of structures that compose the output of the sympathetic system make it possible for these disparate effectors to come together in a coordinated, systemic change.
The sympathetic division of the autonomic nervous system influences the various organ systems of the body through connections emerging from the thoracic and upper lumbar spinal cord. It is referred to as the thoracolumbar system to reflect this anatomical basis. A central neuron in the lateral horn of any of these spinal regions projects to ganglia adjacent to the vertebral column through the ventral spinal roots. The majority of ganglia of the sympathetic system belong to a network of sympathetic chain ganglia that runs alongside the vertebral column. The ganglia appear as a series of clusters of neurons linked by axonal bridges. A diagram that shows the connections of the sympathetic system is somewhat like a circuit diagram that shows the electrical connections between different receptacles and devices (Figure 15.22, wherein the “circuits” of the sympathetic system are intentionally simplified).
An axon from the central neuron that projects to a sympathetic ganglion is referred to as a preganglionic fiber or neuron and represents the output from the central nervous system to the ganglion. Because the sympathetic ganglia are adjacent to the vertebral column, preganglionic sympathetic fibers are relatively short and are myelinated. A postganglionic fiber—the axon from a ganglionic neuron that projects to the target effector—represents the output of a ganglion that directly influences the organ. Compared with the preganglionic fibers, postganglionic sympathetic fibers are long because of the relatively greater distance from the ganglion to the target effector. These fibers are unmyelinated. (Note that the term “postganglionic neuron” may be used to describe the projection from a ganglion to the target. The problem with that usage is that the cell body is in the ganglion, and only the fiber is postganglionic. Typically, the term neuron applies to the entire cell.)
One type of preganglionic sympathetic fiber does not terminate in a ganglion. These are the axons from central sympathetic neurons that project to the adrenal medulla, the interior portion of the adrenal gland. These axons are still referred to as preganglionic fibers, but the target is not a ganglion. The adrenal medulla releases signaling molecules into the bloodstream rather than using axons to communicate with target structures.
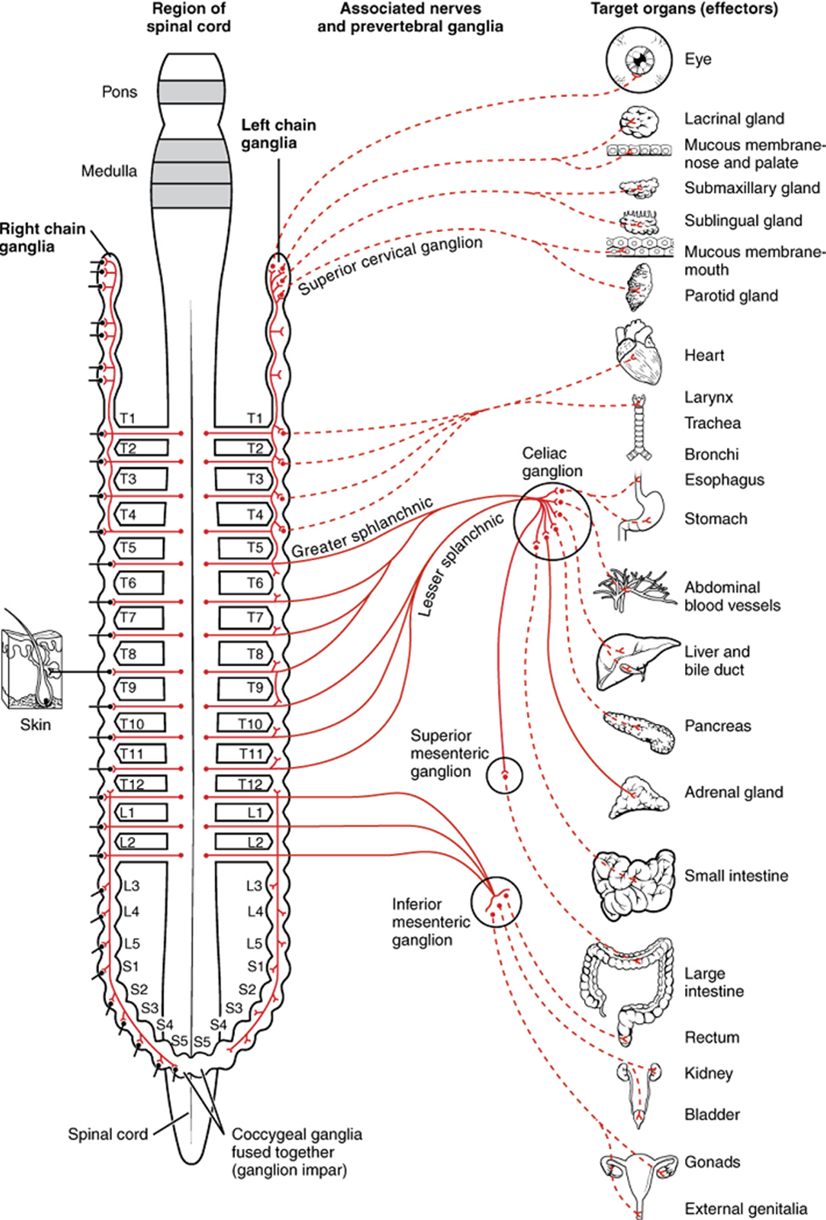
The projections of the sympathetic division of the autonomic nervous system diverge widely, resulting in a broad influence of the system throughout the body. As a response to a threat, the sympathetic system would increase heart rate and breathing rate and cause blood flow to the skeletal muscle to increase and blood flow to the digestive system to decrease. Sweat gland secretion should also increase as part of an integrated response. All of those physiological changes are going to be required to occur together to run away from the hunting lioness, or the modern equivalent. This divergence is seen in the branching patterns of preganglionic sympathetic neurons—a single preganglionic sympathetic neuron may have 10–20 targets. An axon that leaves a central neuron of the lateral horn in the thoracolumbar spinal cord will pass through the white ramus communicans and enter the sympathetic chain, where it will branch toward a variety of targets. At the level of the spinal cord at which the preganglionic sympathetic fiber exits the spinal cord, a branch will synapse on a neuron in the adjacent chain ganglion. Some branches will extend up or down to a different level of the chain ganglia. Other branches will pass through the chain ganglia and project through one of the splanchnic nerves to a collateral ganglion. Finally, some branches may project through the splanchnic nerves to the adrenal medulla. All of these branches mean that one preganglionic neuron can influence different regions of the sympathetic system very broadly by acting on widely distributed organs.
Parasympathetic Division of the Autonomic Nervous System
When not responding to an immediate threat, the parasympathetic system is generally more active than the sympathetic system. Many of the same effectors in the body are innervated by both divisions of the autonomic nervous system, but activation of each division tends to have opposing effects. Sympathetic system activation tends to increase activity in the respiratory, cardiovascular, and musculoskeletal systems while reducing activity in the digestive system. Parasympathetic system activation on the other hand tends to decrease activity in the respiratory, cardiovascular, and musculoskeletal systems while increasing activity in the digestive, urinary, and reproductive systems. Generally speaking, the activity of the many organs that receive input from both systems is dependent on whether neurons of the parasympathetic or sympathetic system are releasing more of their neurotransmitter onto each organ at a given time.
The parasympathetic division of the autonomic nervous system is named because its central neurons are located on either side of the thoracolumbar region of the spinal cord (para- = “beside” or “near”). The parasympathetic system can also be referred to as the craniosacral system (or outflow) because the preganglionic neurons are located in nuclei of the brain stem and the lateral horn of the sacral spinal cord.
The connections, or “circuits,” of the parasympathetic division are similar to the general layout of the sympathetic division with a few specific differences (Figure 15.23). The preganglionic fibers from the cranial region travel in cranial nerves, whereas preganglionic fibers from the sacral region travel in spinal nerves. The targets of these fibers are terminal ganglia, which are located near—or even within—the target organ. The postganglionic fiber projects from the terminal ganglia a short distance to the effector. These ganglia are often referred to as intramural ganglia when they are found within the walls of the target effector or to the specific target tissue within the organ. Comparing the relative lengths of axons in the parasympathetic system, the preganglionic fibers are long, and the postganglionic fibers are short because the ganglia are close to—and sometimes within—the target effectors.
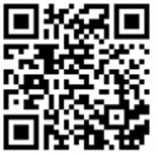
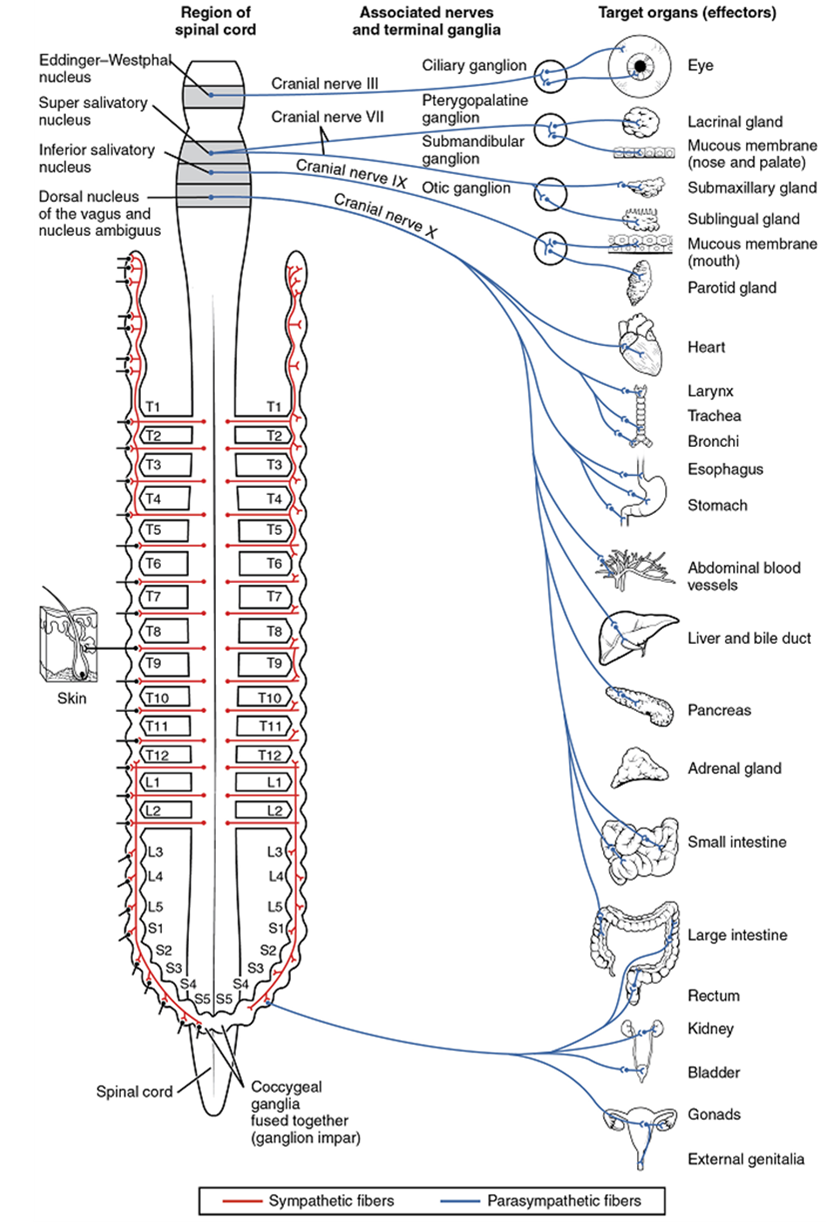
Chemical Signaling in the Autonomic Nervous System
Where an autonomic neuron innervates a target, there is a synapse. The electrical signal of the action potential causes the release of a signaling molecule, which will bind to receptor proteins on the target cell. Synapses of the autonomic system are classified as either cholinergic, meaning that acetylcholine (ACh) is released, or adrenergic, meaning that norepinephrine is released. The terms cholinergic and adrenergic refer not only to the signaling molecule that is released but also to the class of receptors that each binds.
The term adrenergic should remind you of the word adrenaline, which is associated with the fight-or-flight response described at the beginning of the chapter. Adrenaline and epinephrine are two names for the same molecule. The adrenal gland (in Latin, ad- = “on top of”; renal = “kidney”) secretes adrenaline. The ending “-ine” refers to the chemical being derived, or extracted, from the adrenal gland. A similar construction from Greek instead of Latin results in the word epinephrine (epi- = “above”; nephr- = “kidney”). In scientific usage, epinephrine is preferred in the United States, whereas adrenaline is preferred in Great Britain, because “adrenalin” was once a registered, proprietary drug name in the United States. Though the drug is no longer sold, the convention of referring to this molecule by the two different names persists. Similarly, norepinephrine and noradrenaline are two names for the same molecule.
All preganglionic fibers, both sympathetic and parasympathetic, release ACh. The postganglionic parasympathetic fibers also release ACh. Postganglionic sympathetic fibers release norepinephrine, except for fibers that project to sweat glands and to blood vessels associated with skeletal muscles, which release ACh.
Signaling molecules can belong to two broad groups. Neurotransmitters are released at synapses, whereas hormones are released into the bloodstream. These are simplistic definitions, but they can help to clarify this point. Acetylcholine can be considered a neurotransmitter because it is released by axons at synapses. The adrenergic system, however, presents a challenge. Postganglionic sympathetic fibers release norepinephrine, which can be considered a neurotransmitter. But the adrenal medulla releases epinephrine and norepinephrine into circulation, so they should be considered hormones.
Test Your Knowledge
V. Describe the components of a reflex arc and explain how a reflex arc works.
- Describe the events that take place from the moment the knee is tapped to the moment when the leg extends during the patellar reflex, including the role of each of the structures involved.
VI. Describe the function of the autonomic nervous system (ANS) and compare the specific functions of the parasympathetic and sympathetic divisions of the ANS.
- Compare the sympathetic and parasympathetic nervous system based on the:
-
- Physiological situation to which they respond
- Location and neurotransmitter of the central (preganglionic) neuron
- Location and neurotransmitter of the ganglionic neuron
Part 5: Neuronal Signaling
Having looked at the components of nervous tissue and the basic anatomy of the nervous system, next comes an understanding of how nervous tissue is capable of communicating within the nervous system. Before getting to the nuts and bolts of how this works, an illustration of how the components come together will be helpful (summarized in Figure 15.24).
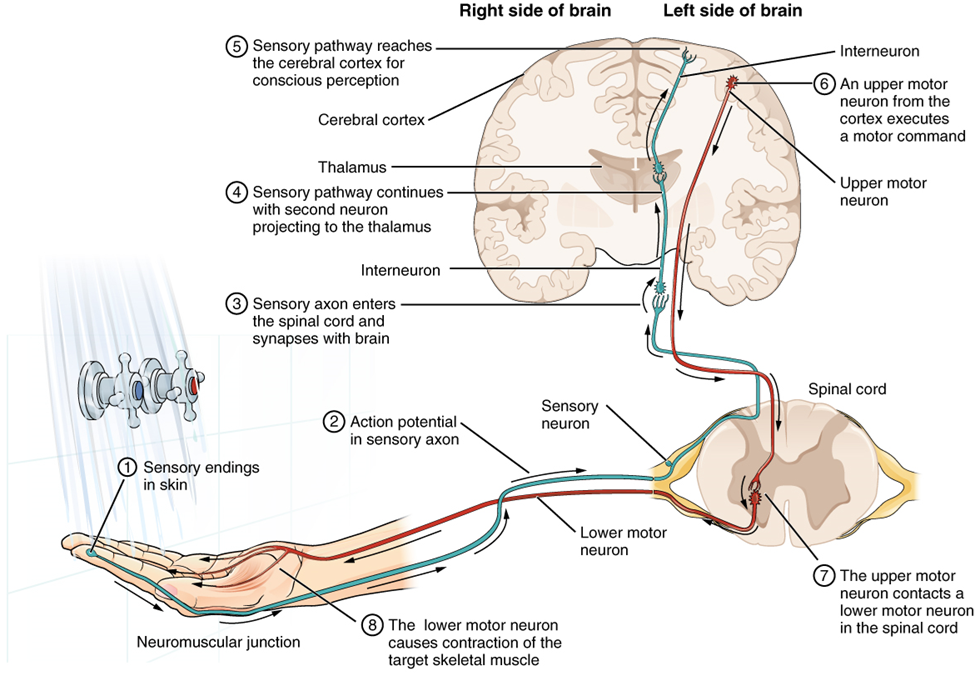
Imagine you are about to take a shower. You have turned on the faucet to start the water as you prepare to get in the shower. After a few minutes, you expect the water to be a temperature that will be comfortable to enter. So you put your hand out into the spray of water. What happens next depends on how your nervous system interacts with the stimulus of the water temperature and what you do in response to that stimulus.
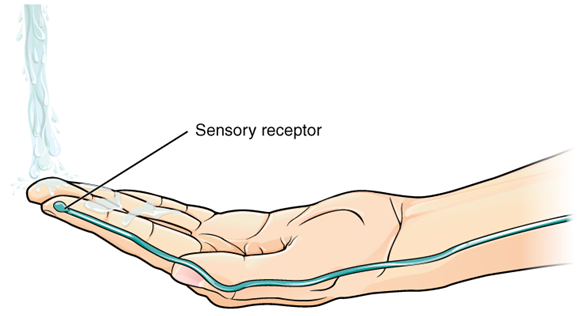
Found in the skin of your fingers or toes is a type of sensory receptor that is sensitive to temperature, called a thermoreceptor. When you place your hand under the shower (Figure 15.25), the cell membrane of the thermoreceptor changes its electrical state (voltage). The amount of change is dependent on the strength of the stimulus (how hot the water is). This is called a graded potential. If the stimulus is strong, the voltage of the cell membrane will change enough to generate an electrical signal that will propagate down the axon.
The voltage at which such a signal is generated is called the threshold, and the resulting electrical signal is called an action potential. In this example, the action potential travels—a process known as propagation—along the axon from the axon hillock to the axon terminals and into the synaptic end bulbs. Propagation is a process by which the action potential is constantly re-created along small stretches of membrane, appearing to “travel.” When this signal reaches the end bulbs, it causes the release of a signaling molecule called a neurotransmitter.
The neurotransmitter diffuses across the short distance of the synapse and binds to a receptor protein on the target neuron. When the molecular signal binds to the receptor, the cell membrane of the target neuron changes its electrical state, and a new graded potential begins. If that graded potential is strong enough to reach threshold, the second neuron generates an action potential at its axon hillock. The target of this neuron is another neuron in the thalamus of the brain, the part of the central nervous system that acts as a relay for sensory information. At another synapse, a neurotransmitter is released and binds to its receptor. The thalamus then sends the sensory information to the cerebral cortex, the outermost layer of gray matter in the brain, where conscious perception of the water temperature begins. Within the cerebral cortex, information is processed among many neurons, integrating the stimulus of the water temperature with other sensory stimuli, with your emotional state (you just aren’t ready to wake up; the bed is calling to you), and memories (perhaps of the lab notes you have to study before a quiz). Finally, a plan is developed about what to do, whether that is to turn the temperature up, turn the whole shower off and go back to bed, or step into the shower. To do any of these things, the cerebral cortex has to send a command out to your body to move muscles (Figure 15.26).
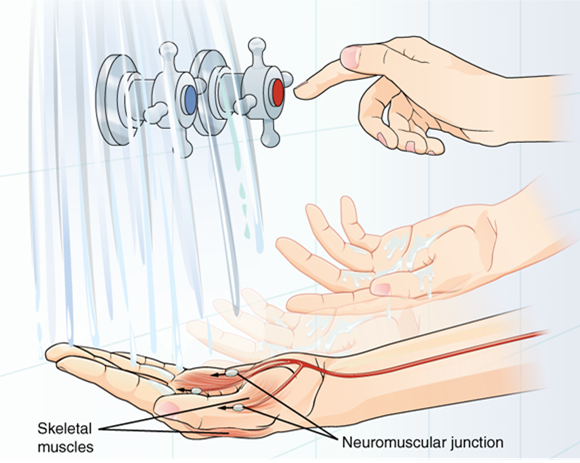
A region of the cortex is specialized for sending signals down to the spinal cord for movement. The upper motor neuron is in this region, called the primary motor cortex, which has an axon that extends all the way down the spinal cord. At the level of the spinal cord at which this axon makes a synapse, a graded potential occurs in the cell membrane of a lower motor neuron. This second motor neuron is responsible for causing muscle fibers to contract. In the manner described in the chapter on muscle tissue, an action potential propagates along the motor neuron axon into the periphery. The axon terminates on muscle fibers at the neuromuscular junction. Acetylcholine is released at this specialized synapse, which causes the muscle action potential to begin, following a large potential known as an end plate potential. When the lower motor neuron excites the muscle fiber, it contracts. All of this occurs in a fraction of a second, but this story is the basis of how the nervous system functions.
Ion Channels and the Resting Membrane Potential
The functions of the nervous system—sensation, integration, and response—depend on the functions of the neurons underlying these pathways. To understand how neurons are able to communicate, it is necessary to describe the role of an excitable membrane in generating these signals. The basis of this communication is the action potential, which demonstrates how changes in the membrane can constitute a signal. (The way these signals work in more variable circumstances involves graded potentials.)
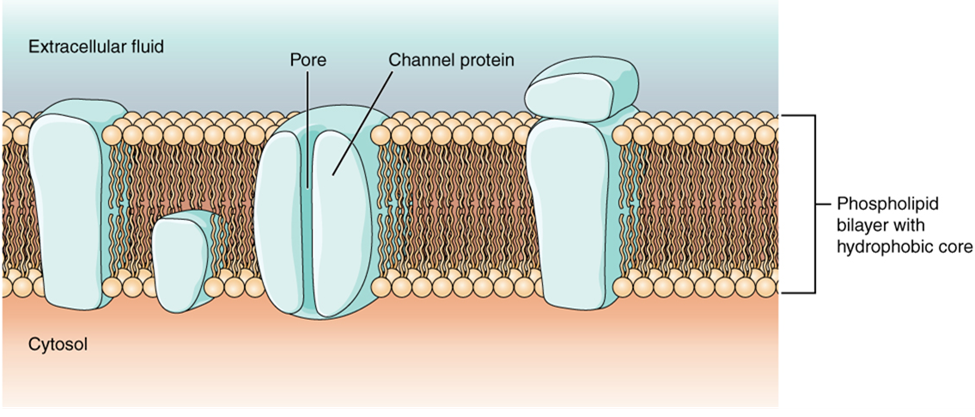
Cells in the body make use of charged particles, ions, to build up a charge across the cell membrane. The cell membrane regulates ion movement between the extracellular fluid and cytosol. As you learned in Chapter 6, the cell membrane is primarily responsible for regulating what can cross the membrane. The cell membrane is a phospholipid bilayer, so only substances that can pass directly through the hydrophobic core can diffuse through without assistance. Charged particles, which are hydrophilic by definition, cannot pass through the cell membrane without assistance (Figure 15.27). Transmembrane proteins, specifically channel proteins, make this possible. Several passive ion channels, as well as active transport pumps, are necessary to generate a membrane potential and an action potential. Ion channels are pores that allow specific charged particles to cross the membrane in response to an existing concentration gradient.
Of special interest is the carrier protein referred to as the sodium/potassium pump that actively moves sodium ions (Na+) out of a cell and potassium ions (K+) into a cell, thus regulating ion concentration on both sides of the cell membrane. The sodium/potassium pump requires energy in the form of adenosine triphosphate (ATP), so it is also referred to as an ATPase. As was explained in Chapter 7, the concentration of Na+ is higher outside the cell than inside, and the concentration of K+ is higher inside the cell than outside. That means that this pump is moving the ions against the concentration gradients for sodium and potassium, which is why it requires energy. The only purpose of this pump is to maintain these concentration gradients.
Ion channels typically do not allow ions to diffuse across the membrane. Most are opened by certain events, meaning the channels are gated.
A ligand-gated channel opens because a signaling molecule, a ligand, binds to the extracellular region of the channel. This type of channel is also known as an ionotropic receptor because when the ligand, typically a neurotransmitter in the nervous system, binds to the protein, ions cross the membrane, changing membrane potential (Figure 15.28).
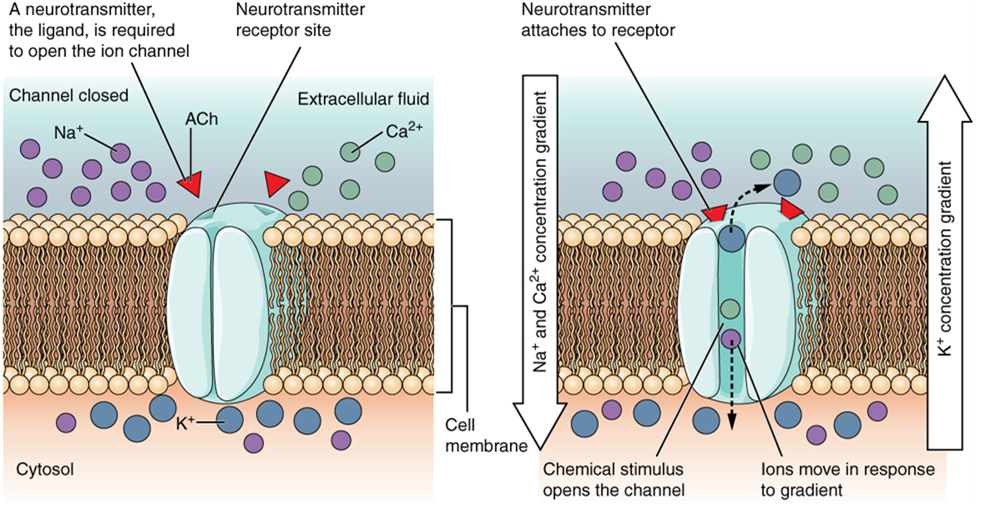
A mechanically gated channel opens because of a physical distortion of the cell membrane. Many channels associated with the sense of touch (somatosensation) are mechanically gated. For example, as pressure is applied to the skin, these channels open and allow ions to enter the cell. Similar to this type of channel would be the channel that opens on the basis of temperature change, as in testing the water in the shower (Figure 15.29).
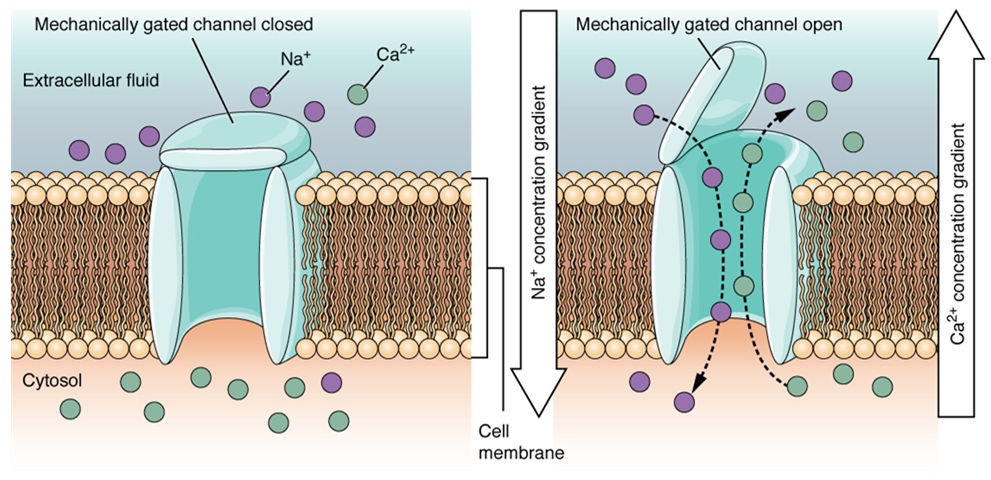
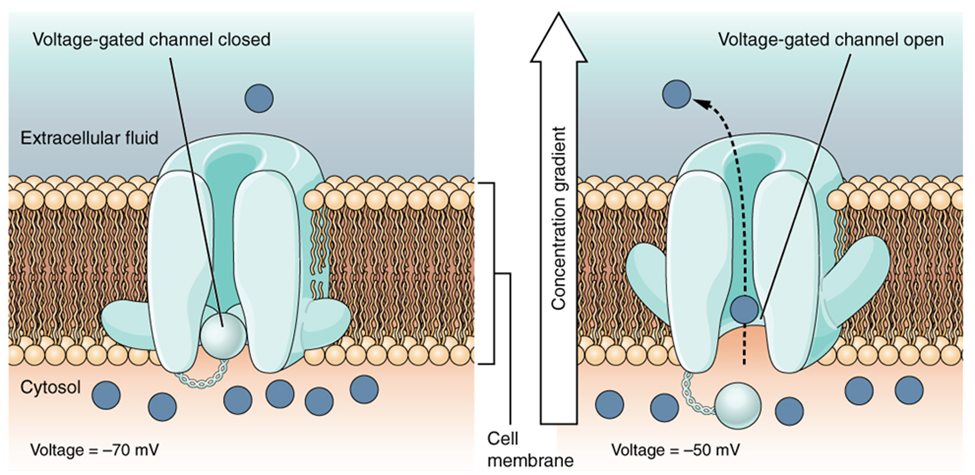
A voltage-gated channel is a channel that responds to changes in the electrical properties of the membrane in which it is embedded. Normally, the inner portion of the membrane is at a negative voltage. When that voltage becomes less negative, the channel begins to allow ions to cross the membrane (Figure 15.30).
A leakage channel is randomly gated, meaning that it opens and closes at random, hence the reference to leaking. There is no actual event that opens the channel; instead, it has an intrinsic rate of switching between the open and closed states. Leakage channels contribute to the resting voltage of the excitable membrane (Figure 15.31).
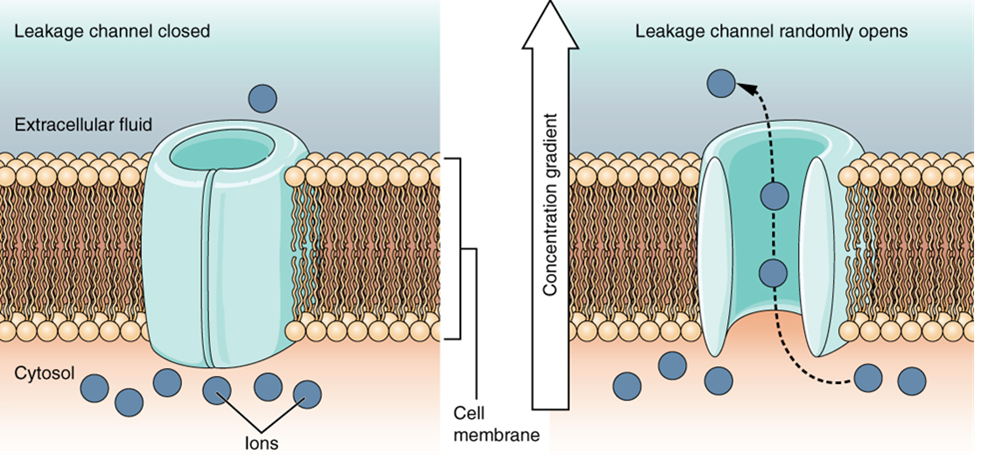
The electrical state of the cell membrane can have several variations. These are all variations in the membrane potential. A potential is a distribution of charge across the cell membrane, measured in millivolts (mV). The standard is to compare the inside of the cell relative to the outside, so the membrane potential is a value representing the charge on the intracellular side of the membrane relative to the outside (Figure 15.32).
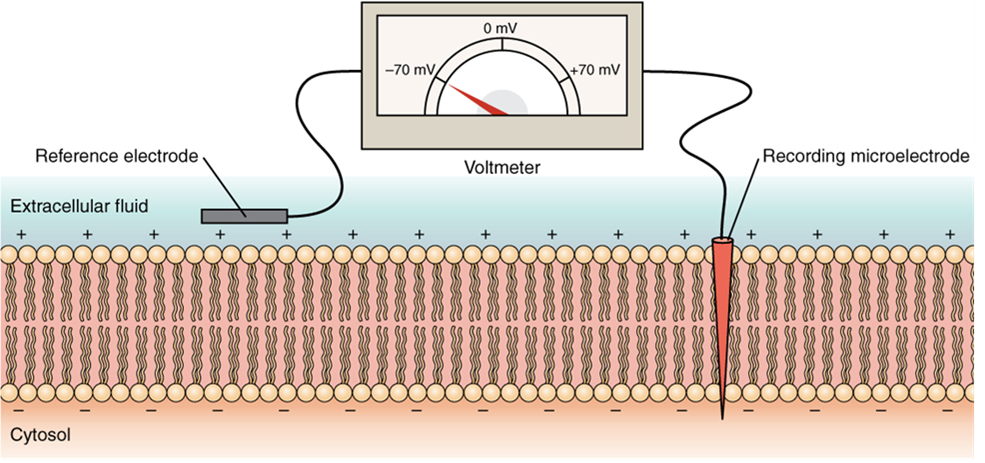
The concentration of ions in extracellular and intracellular fluids is largely balanced, with a net neutral charge. However, a slight difference in charge occurs right at the membrane surface, both internally and externally. It is the difference in this very limited region that has all the power in neurons (and muscle cells) to generate electrical signals, including action potentials.
Before these electrical signals can be described, the resting state of the membrane must be explained. When the cell is at rest, and the ion channels are closed (except for leakage channels, which randomly open), ions are distributed across the membrane in a very predictable way. The concentration of Na+ outside the cell is 10 times greater than the concentration inside. Also, the concentration of K+ inside the cell is greater than outside. The cytosol contains a high concentration of anions in the form of phosphate ions and negatively charged proteins. Large anions are a component of the inner cell membrane, including specialized phospholipids and proteins associated with the inner leaflet of the membrane (leaflet is a term used for one side of the lipid bilayer membrane). The negative charge is localized in the large anions.
With the ions distributed across the membrane at these concentrations, the difference in charge is measured at -70 mV for most cells, the value described as the resting membrane potential. The exact value measured for the resting membrane potential varies between cells, but -70 mV is the most commonly recorded value. This voltage would actually be much lower except for the contributions of some important proteins in the membrane. Leakage K+ channels allow K+ to slowly move out of the cells. To a much lesser extent, leakage Na+ channels allow Na+ to slowly move into the cell. The constant activity of the Na+/K+ pump maintains the ion gradients. This may appear to be a waste of energy, but this pump plays a vital role in maintaining the membrane potential.
Generation of an Action Potential
Resting membrane potential describes the steady state of the cell, which is a dynamic process that is balanced by ion leakage and ion pumping. Without any outside influence, it will not change. To get an electrical signal started, the membrane potential has to change.
This starts with a channel opening for Na+ in the membrane. Because the concentration of Na+ is higher outside the cell than inside the cell by a factor of 10, ions will rush into the cell that are driven largely by the concentration gradient. Because sodium is a positively charged ion, it will change the relative voltage immediately inside the cell relative to immediately outside. The resting potential is the state of the membrane at a voltage of -70 mV, so the sodium cation entering the cell will cause it to become less negative. This is known as depolarization, meaning the membrane potential moves toward zero.
The concentration gradient for Na+ is so strong that it will continue to enter the cell even after the membrane potential has become zero so that the internal voltage immediately around the pore begins to become positive. The electrical gradient also plays a role, as negative proteins below the membrane attract the sodium ion. The membrane potential will reach +30 mV as a result of sodium entering the cell.
As the membrane potential reaches +30 mV, other voltage-gated channels are opening in the membrane. These channels are specific for the potassium ion. A concentration gradient acts on K+, as well. As K+ starts to leave the cell, taking a positive charge with it, the membrane potential begins to move back toward its resting voltage. This is called repolarization, meaning that the membrane voltage moves back toward the -70 mV value of the resting membrane potential.
Repolarization returns the membrane potential to the -70 mV value that indicates the resting potential, but it actually overshoots that value. Potassium ions reach equilibrium when the membrane voltage is below -70 mV, so a period of hyperpolarization occurs while the K+ channels are open. Those K+ channels are slightly delayed in closing, accounting for this short overshoot.
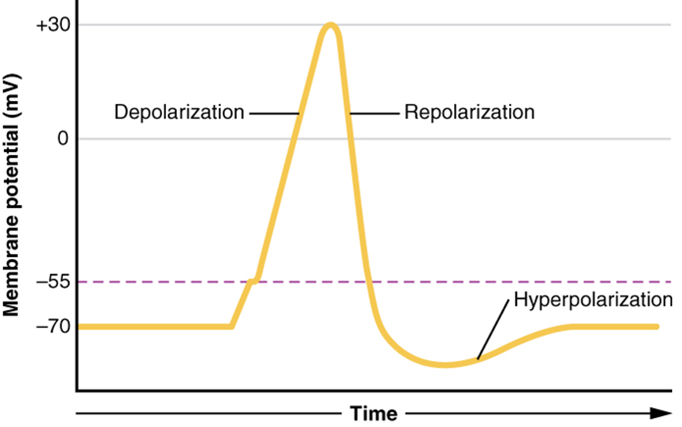
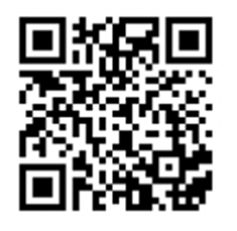
What has been described above is the action potential, which is presented as a graph of voltage over time (Figure 15.33). It is the electrical signal that nervous tissue generates for communication. The change in the membrane voltage from -70 mV at rest to +30 mV at the end of depolarization is a 100-mV change. That can also be written as a 0.1-V change. To put that value in perspective, think about a battery. An AA battery that you might find in a television remote has a voltage of 1.5 V, or a 9-V battery (the rectangular battery with two posts on one end) is, obviously, 9 V. The change seen in the action potential is one or two orders of magnitude less than the charge in these batteries. In fact, the membrane potential can be described as a battery. A charge is stored across the membrane that can be released under the correct conditions. A battery in your remote has stored a charge that is “released” when you push a button.
The question is, now, what initiates the action potential? The description above conveniently glosses over that point. But it is vital to understanding what is happening. The membrane potential will stay at the resting voltage until something changes. The description above just says that a Na+ channel opens. Now, to say “a channel opens” does not mean that one individual transmembrane protein changes. Instead, it means that one kind of channel opens. There are a few different types of channels that allow Na+ to cross the membrane. A ligand-gated Na+ channel will open when a neurotransmitter binds to it, and a mechanically gated Na+ channel will open when a physical stimulus affects a sensory receptor (like pressure applied to the skin compresses a touch receptor). Whether it is a neurotransmitter binding to its receptor protein or a sensory stimulus activating a sensory receptor cell, some stimulus gets the process started. Sodium starts to enter the cell, and the membrane becomes more positive.
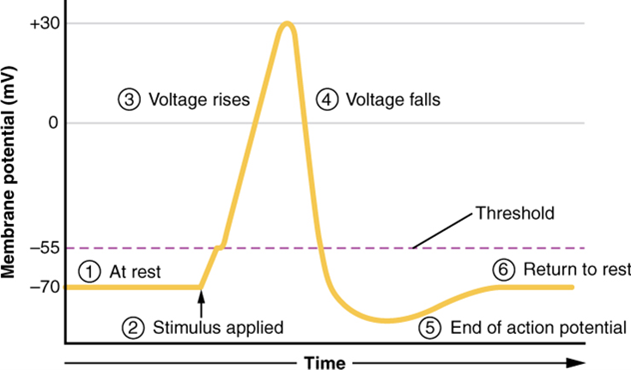
A third type of channel that is an important part of depolarization in the action potential is the voltage-gated Na+ channel. The channels that start depolarizing the membrane because of a stimulus help the cell to depolarize from -70 mV to -55 mV. A membrane potential of -55 mV is known as “threshold” for most cells. Once the membrane reaches that voltage, the voltage-gated Na+ channels open. Any depolarization that does not change the membrane potential to -55 mV or higher will not reach threshold and thus will not result in an action potential. Also, any stimulus that depolarizes the membrane to -55 mV or beyond will cause a large number of channels to open, and an action potential will be initiated.
Because of the threshold, the action potential can be likened to a digital event—it either happens or it does not. If the threshold is not reached, then no action potential occurs. If depolarization reaches -55 mV, then the action potential continues and runs all the way to +30 mV, at which point K+ causes repolarization, including the hyperpolarizing overshoot. Also, those changes are the same for every action potential, which means that once the threshold is reached, the exact same thing happens. A stronger stimulus, which might depolarize the membrane well past the threshold, will not make a “bigger” action potential. Action potentials are “all or none.” Either the membrane reaches the threshold and everything occurs, as described above, or the membrane does not reach the threshold and nothing else happens. All action potentials peak at the same voltage (+30 mV), so one action potential is not bigger than another. Stronger stimuli will initiate multiple action potentials more quickly, but the individual signals are not bigger. Thus, for example, you will not feel a greater sensation of pain, or have a stronger muscle contraction, due to the size of the action potential because they are not different sizes. Only a change in action potential frequency allows us to perceive stimuli in terms of magnitude.
As we have seen, the depolarization and repolarization of an action potential are dependent on two types of channels (the voltage-gated Na+ channel and the voltage-gated K+ channel). The voltage-gated Na+ channel actually has two gates. One is the activation gate, which opens when the membrane potential reaches -55 mV. The other gate is the inactivation gate, which closes after a specific period of time—on the order of a fraction of a millisecond. When a cell is at rest, the activation gate is closed, and the inactivation gate is open. However, when the threshold is reached, the activation gate opens, allowing Na+ to rush into the cell. Timed with the peak of depolarization, the inactivation gate closes. Once this occurs, the channel is said to be “inactivated.” Think of this as a locked door. During repolarization, no more sodium can enter the cell. When the membrane potential returns to -55 mV, the activation gate closes. After that, the inactivation gate re-opens, making the channel ready to start the whole process over again. Think of this as a door being closed but unlocked.
The voltage-gated K+ channel has only one gate, which is sensitive to a membrane voltage of -50 mV. However, it does not open or close as quickly as the voltage-gated Na+ channel does. It might take a fraction of a millisecond for the channel to open once that voltage has been reached. The timing of this coincides exactly with when the Na+ flow peaks, so voltage-gated K+ channels open just as the voltage-gated Na+ channels are being inactivated. As the membrane potential repolarizes and the voltage passes -50 mV again, the channel closes—again, with a little delay. Potassium continues to leave the cell for a short while, and the membrane potential becomes more negative than it was at rest, resulting in the hyperpolarizing overshoot. Then the K+ channel closes again and the membrane can return to the resting potential due to the ongoing activity of the nongated channels and the Na+/K+ pump. All of this takes place within approximately 2 milliseconds (Figure 15.34). While an action potential is in progress, another one cannot be initiated. That effect is referred to as the refractory period.
Propagation of Action Potentials
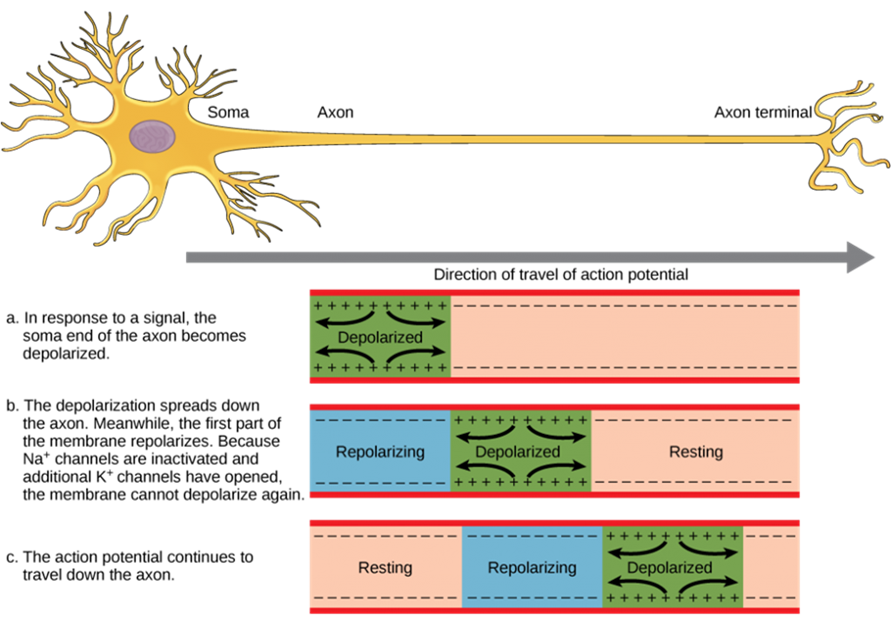
The action potential is initiated at the beginning of the axon, at what is called the initial segment, or axon hillock. There is a high density of voltage-gated Na+ channels so that rapid depolarization can take place here. Going down the length of the axon, the action potential is propagated because more voltage-gated Na+ channels are opened as the depolarization spreads. This spreading occurs because Na+ enters through its channel and passively spreads along the inside of the cell membrane. As the Na+ moves, or flows, a short distance along the cell membrane, its positive charge depolarizes a little more of the cell membrane. As that depolarization spreads, new voltage-gated Na+ channels open and more ions rush into the cell, spreading the depolarization a little farther (Figure 15.35).
Because voltage-gated Na+ channels are inactivated at the peak of the depolarization, they cannot be opened again for a brief time—the absolute refractory period. Because of this, depolarization spreading back toward previously opened channels has no effect. The action potential must propagate toward the axon terminals; as a result, the polarity of the neuron is maintained, as mentioned above. Thus, the absolute refractory period ensures that the action potential can only propagate toward the axon terminal.
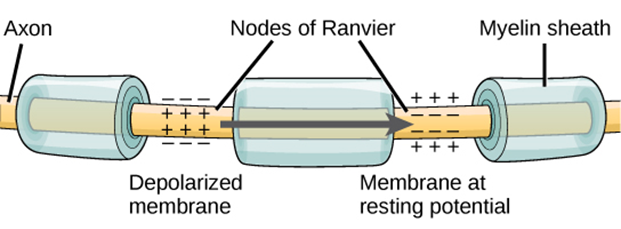
Propagation, as described above, applies to unmyelinated axons. When myelination is present, the action potential propagates differently (Figure 15.36). Sodium ions that enter the cell at the initial segment start to spread along the length of the axon segment, but there are no voltage-gated Na+ channels until the first node of Ranvier. Because there is not a constant opening of these channels along the axon segment, the depolarization spreads at an optimal speed. The distance between nodes (1–3 mm) is the optimal distance to keep the membrane still depolarized above threshold at the next node. As Na+ spreads along the inside of the membrane of the axon segment, the charge starts to dissipate. If the nodes were any farther down the axon, that depolarization would have fallen off too much for voltage-gated Na+ channels to be activated at the next node of Ranvier. If the nodes were any closer together, the speed of propagation would be slower.
Propagation along an unmyelinated axon is referred to as continuous conduction; along the length of a myelinated axon, it is saltatory conduction. Continuous conduction is slow because there are always voltage-gated Na+ channels opening, and more and more Na+ is rushing into the cell. Saltatory conduction is faster because the action potential basically jumps from one node to the next (saltare = “to leap”), and the new influx of Na+ renews the depolarized membrane. Along with the myelination of the axon, the diameter of the axon can influence the speed of conduction. Consider the speed of water through a fire hose compared to that of an ordinary garden hose. Similarly, Na+-based depolarization spreads faster down a wide axon than down a narrow one. This concept is known as internal resistance and is generally true for electrical wires or plumbing, just as it is true for axons.
Neurotransmission
The electrical changes taking place within a neuron, as described in the previous section, are similar to a light switch being turned on. A stimulus starts the depolarization, but the action potential runs on its own once a threshold has been reached. The question is now, “What flips the light switch on?” Temporary changes to the cell membrane voltage can result from neurons receiving information from the environment, or from the action of one neuron to another. These special types of potentials influence a neuron and determine whether an action potential will occur. Many of these transient signals originate at the synapse, the connection between electrically active cells.
There are two types of synapses: chemical synapses and electrical synapses. In a chemical synapse, a chemical signal—namely, a neurotransmitter—is released from one cell, and it causes a change in the other cell. In an electrical synapse, there is a direct connection between the two cells so that ions can pass directly from one cell to the next. If one cell is depolarized in an electrical synapse, the joined cell also depolarizes because the ions pass between the cells. Think about two rooms of a hotel suite connected by a door that is always open. Chemical synapses involve the transmission of chemical information from one cell to the next. This section will concentrate on the chemical synapse.
An example of a chemical synapse is the neuromuscular junction described in Chapter 14, Muscle Physiology. In the nervous system, there are many more synapses that are essentially the same as the neuromuscular junction. All synapses have common characteristics, which can be summarized in this list:
- presynaptic element
- neurotransmitter (packaged in vesicles)
- synaptic cleft
- receptor proteins
- postsynaptic element
- neurotransmitter elimination or re-uptake
Synaptic transmission (or neurotransmission) takes place through the following steps (Figure 15.37):
- An action potential reaches the axon terminal.
- The change in voltage causes voltage-gated Ca2+ channels in the membrane of the synaptic end bulb to open.
- The concentration of Ca2+ increases inside the end bulb, and Ca2+ ions associate with proteins on the outer surface of neurotransmitter vesicles, facilitating the merging of the vesicles with the presynaptic membrane. The neurotransmitter is then released through exocytosis into the small gap between the cells, known as the synaptic cleft.
- Once in the synaptic cleft, the neurotransmitter diffuses the short distance to the postsynaptic membrane and can interact with neurotransmitter receptors. Receptors are specific for the neurotransmitter, and the two fit together like a key and lock. Each neurotransmitter binds to its receptor and will not bind to receptors for other neurotransmitters, making the binding a specific chemical event.
- The interaction of the neurotransmitter with the receptor can result in depolarization or hyperpolarization of the postsynaptic cell membrane, leading to excitation of the postsynaptic cell (and possibly the generation of a new action potential) or inhibition, respectively. The terms excitation and inhibition describe the likelihood that the change in membrane potential will produce an action potential. Thus, positive changes bring the membrane potential closer to threshold, making an action potential more likely, and are referred to as excitatory. Inhibitory changes do the opposite.
- The neurotransmitter is removed from the synaptic cleft by diffusion, due to the action of enzymes that chemically break it down, or by transporters in the presynaptic cell membrane.
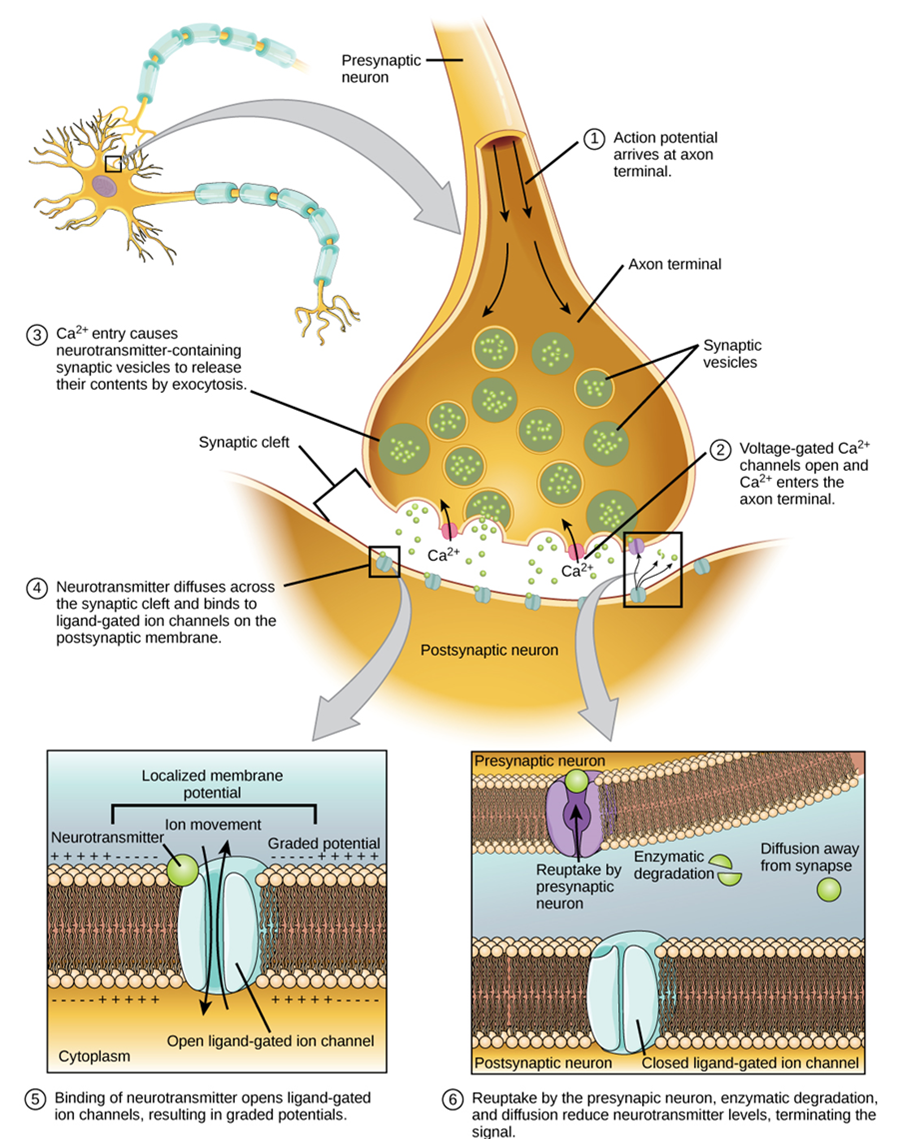
Neurotransmitter Systems
There are several systems of neurotransmitters that are found at various synapses in the nervous system. In this course, you are not required to know all the neurotransmitters but only to be able to provide one example of a neurotransmitter from each of the systems below.
- Amino acids: This includes glutamate (Glu), GABA (gamma-aminobutyric acid, a derivative of glutamate), and glycine (Gly).
- Biogenic amines: This is a group of neurotransmitters that are enzymatically made from amino acids. For example, the neurotransmitter serotonin is made from tryptophan. Other biogenic amines are made from tyrosine and include dopamine, norepinephrine, and epinephrine. The chemical epinephrine (epi- = “on”; “-nephrine” = kidney) is also known as adrenaline (renal = “kidney”), and norepinephrine is sometimes referred to as noradrenaline. The adrenal gland produces epinephrine and norepinephrine to be released into the bloodstream as hormones.
- Cholinergic system: This is the system based on acetylcholine. This includes the neuromuscular junction as an example of a cholinergic synapse, but cholinergic synapses are also found in other parts of the nervous system. They are located in the autonomic nervous system, as well as distributed throughout the brain.
- Neuropeptides: These are neurotransmitter molecules made up of chains of amino acids connected by peptide bonds. This is what a protein is, but the term protein implies a certain length to the molecule. Some neuropeptides are quite short, such as met-enkephalin, which is five amino acids long. Others are long, such as beta-endorphin, which is 31 amino acids long. Neuropeptides are often released at synapses in combination with another neurotransmitter, and they often act as hormones in other systems of the body, such as vasoactive intestinal peptide (VIP) or substance P.
The effect of a neurotransmitter on the postsynaptic element is entirely dependent on the receptor protein. First, if there is no receptor protein in the membrane of the postsynaptic element, then the neurotransmitter has no effect. The depolarizing or hyperpolarizing effect is also dependent on the receptor. For example, when acetylcholine binds to a type of receptor called nicotinic receptor, the postsynaptic cell is depolarized. This is because the receptor is a cation channel, and positively charged Na+ will rush into the cell. However, when acetylcholine binds to another type of receptor called muscarinic receptor, of which there are several variants, it might cause depolarization or hyperpolarization of the target cell.
On the other hand, the amino acid neurotransmitters, glutamate, glycine, and GABA, are almost exclusively associated with just one effect. Glutamate is considered an excitatory amino acid but only because Glu receptors in the adult cause depolarization of the postsynaptic cell. Glycine and GABA are considered inhibitory amino acids, again because their receptors cause hyperpolarization..
Test Your Knowledge
VII. Describe the resting membrane potential of a neuron and explain how it is maintained.
- Describe the gating mechanism of ligand-gated, voltage-gated, mechanically-gated and leakage ion channels.
- What is the typical resting membrane potential of an animal cell, and what factors contribute to it?
VIII. Explain how a neuronal action potential is generated.
- Draw a fully annotated figure plotting membrane potential vs. time as an action potential passes a specific location in an axon’s membrane. Include in your annotations labels explaining the main mechanisms that underlie each shift in membrane potential.
IX. Explain how neuronal action potentials travel down the axon.
- Compare the mechanism by which nerve impulses are conducted in unmyelinated and myelinated axons.
X. Explain the process of neurotransmission, and name three different neurotransmitters.
- Create an annotated diagram (or series of diagrams) showing how neurons communicate with each other:
- Describe the mechanism by which an action potential travels from the cell body to the axon terminals of a neuron.
- Describe the mechanisms that return a neuron to its resting state (resting membrane potential) once an action potential has passed.
- Describe the intracellular events that occur in a neuron once an action potential reaches a synaptic end bulb.
- Describe how an excitatory neurotransmitter causes an action potential to be produced in a postsynaptic cell.
- Name at least three specific neurotransmitters: one from the cholinergic system, one amino acid that acts as a neurotransmitter, and one neuropeptide.
- What factor(s) determines whether a neurotransmitter has an excitatory or inhibitory effect on a cell exposed to that neurotransmitter?
Practice
For the following questions, click the correct answer choice.
Image Descriptions
Figure 15.1 Image description: The nervous system is composed of two major parts, or subdivisions, the central nervous system (CNS) and the peripheral nervous system (PNS). The CNS includes the brain and spinal cord. The brain is the body’s “control center.” The CNS has various centers located within it that carry out the sensory, motor, and integration of data. These centers can be subdivided to Lower Centers (including the spinal cord and brain stem) and Higher Centers, communicating with the brain via effectors. The PNS is a vast network of spinal and cranial nerves that are linked to the brain and the spinal cord. It contains sensory receptors, which help in processing changes in the internal and external environment. This information is sent to the CNS via afferent sensory nerves. The PNS is then subdivided into the autonomic nervous system and the somatic nervous system. The autonomic has involuntary control of internal organs, blood vessels, and smooth and cardiac muscles. The somatic has voluntary control of skin, bones, joints, and skeletal muscle. The two systems function together by way of nerves from the PNS entering and becoming part of the CNS, and vice versa. [Return to image.]
Figure 15.5 Image description: The brain (CNS) is responsible for the perception and processing of sensory stimuli (sensory/autonomic), execution of voluntary motor responses (sensory), and regulation of homeostatic mechanisms (autonomic). Nerves (PNS) contain fibers of sensory and motor neurons (sensory/autonomic). The enteric system, located in the digestive tract, (ENS) is responsible for autonomous functions and can operate independently of the brain and spinal cord. The spinal Cord (CNS) contains initiation of reflexes from the ventral horn (somatic) and lateral horn (autonomic) gray matter and pathways for sensory and motor functions between periphery and the brain (somatic/autonomic). Ganglia (PNS) is responsible for the reception of sensory stimuli by dorsal and cranial ganglia (sensory/somatic) and relay of motor responses by autonomic ganglia (autonomic). [Return to image.]
Figure 15.6 Image description: Parts of a Neuron. An neuron is shown to comprised of four distinct parts or regions: dendrite, cell body, axon, and synapse. The dendrite is the receiving end of the neurons that has many inputs that resemble the branches of a tree feeding into a trunk. Some dendrites of the neuron appear to contact the synapse of another neuron. The cell body is a swollen area adjacent to the dendrite that contains the nucleus of the neuron cell and functions in assessing the dendrite’s inputs. A single axon extends away from the cell body like a string and carries nervous impulses towards the synapse; a material called myelin appears to cover discontinuously parts of the axon but no other part of the neuron. Finally, the synapse has short extensions reaching towards another cell. [Return to image.]
Figure 15.8 Image description: Some neurons have atypical morphology that lends them special names. Pyramidal cells have a conical, rather than swollen, cell body. Purkinje fibers have a massive input of dendrites that resemble a huge antler. Other neurons, such as olfactory neurons are only found in association with another structure; in this case, olfactory neurons are found in association with the olfactory epithelium of the nose. [Return to image.]
Figure 15.18 Image description: Meningeal Layers of Superior Sagittal Sinus. The layers of the meninges in the longitudinal fissure of the superior sagittal sinus are shown, with the dura mater adjacent to the inner surface of the cranium, the pia mater adjacent to the surface of the brain, and the arachnoid and subarachnoid space between them. An arachnoid villus is shown emerging into the dural sinus to allow CSF to filter back into the blood for drainage. [Return to image.]
Figure 15.19 Image description: Cerebrospinal fluid (CSF) is a clear, colorless bodily fluid that occupies the subarachnoid space and the ventricular system around and inside the brain and spinal cord. The CSF occupies the space between the arachnoid mater (the middle layer of the brain cover, the meninges) and the pia mater (the layer of the meninges closest to the brain). It constitutes the content of all intracerebral ventricles, cisterns, and sulci (singular sulcus), as well as the central canal of the spinal cord. It acts as a cushion or buffer for the cortex, providing a basic mechanical and immunological protection for the brain inside the skull and serving a vital function in the cerebral autoregulation of cerebral blood flow. [Return to image.]
Figure 15.20 Image description: The dorsal root ganglia are collections of the cell bodies of sensory neurons located lateral to the spinal cord emerging at each spinal level. They are responsible for conveying various sensory stimuli, including pain, touch, vibration, proprioception, and temperature, from the peripheral nervous system to the central nervous system. [Return to image.]
Figure 15.21 Image description: Knee-jerk reflex, sudden kicking movement of the lower leg in response to a sharp tap on the patellar tendon, which lies just below the kneecap. One of the several positions that a subject may take for the test is to sit with knees bent and with one leg crossed over the other so that the upper foot hangs clear of the floor. The sharp tap on the tendon slightly stretches the quadriceps, the complex of muscles at the front of the upper leg. In reaction, these muscles contract, and the contraction tends to straighten the leg in a kicking motion. Exaggeration or absence of the reaction suggests that there may be damage to the central nervous system. The knee jerk can also be helpful in recognizing thyroid disease. [Return to image.]
Figure 15.22 Image description: To respond to a threat—to fight or to run away—the sympathetic system causes diverse effects as many different effector organs are activated together for a common purpose. More oxygen needs to be inhaled and delivered to the skeletal muscle. The respiratory, cardiovascular, and musculoskeletal systems are all activated together. Additionally, sweating keeps the excess heat that comes from muscle contraction from causing the body to overheat. The digestive system shuts down so that blood is not absorbing nutrients when it should be delivering oxygen to skeletal muscles. To coordinate all these responses, the connections in the sympathetic system diverge from a limited region of the central nervous system (CNS) to a wide array of ganglia that project to the many effector organs simultaneously. The complex set of structures that compose the output of the sympathetic system make it possible for these disparate effectors to come together in a coordinated, systemic change. The sympathetic division of the autonomic nervous system influences various organ systems of the body through connections emerging from the first thoracic (T1) and second lumbar (L2) spinal segments. It is referred to as the thoracolumbar system to reflect this anatomical basis. Sympathetic preganglionic neurons are located in the lateral horns of any of these spinal regions and project to ganglia adjacent to the vertebral column through the ventral roots of the spinal cord. [Return to image.]
Figure 15.23 Image description: The parasympathetic nervous system (PSNS) is a division of the autonomic nervous system (ANS) that controls the activity of the smooth and cardiac muscles and glands. It works in synergy with the sympathetic nervous system (SNS), which complements the PSNS activity. The parasympathetic nervous system is also called the craniosacral division of the ANS, as its central nervous system components are located within the brain and the sacral portion of the spinal cord. The functions of the PNS are commonly described as the “rest and digest” response, since it is involved in slowing down the heart rate, relaxing the sphincter muscles in the gastrointestinal and urinary tracts, and increasing intestinal and gland activity. The final result is conserving energy and regulating basic bodily functions such as digestion and urination. It is contrasted to the sympathetic nervous system, which is described as the “fight and flight” response that occurs in stressful situations and has mainly opposite functions. [Return to image.]
Figure 15.27 Image description: Proteins that extend all the way across the membrane are called transmembrane proteins. The portions of an integral membrane protein found inside the membrane are hydrophobic, while those that are exposed to the cytoplasm or extracellular fluid tend to be hydrophilic. [Return to image.]
Figure 15.28 Image description: The prototypic ligand-gated ion channel is the nicotinic acetylcholine receptor. It consists of a pentamer of protein subunits (typically ααβγδ), with two binding sites for acetylcholine (one at the interface of each alpha subunit). Allow sodium, potassium, and calcium to cross. [Return to image.]
Figure 15.29 Image description: Mechanically-gated ion channels are proteins found in eukaryotic and prokaryotic cell membranes that open in response to mechanical stress. Tension, compression, swelling, and shear stress can alter the conformation of the protein, opening a transmembrane channel that allows the passage of ions for signal transmission. In eukaryotes, mechanically-gated channels are distributed in several regions like the neurons, lungs, skin, bladder, and heart, where they play critical roles in numerous physiological and pathophysiological processes. [Return to image.]
Figure 15.30 Image description: Voltage-gated ion channels are a class of transmembrane proteins that form ion channels that are activated by changes in the electrical membrane potential near the channel. The membrane potential alters the conformation of the channel proteins, regulating their opening and closing. Cell membranes are generally impermeable to ions, thus they must diffuse through the membrane through transmembrane protein channels. They have a crucial role in excitable cells such as neuronal and muscle tissues, allowing a rapid and coordinated depolarization in response to triggering voltage change. Found along the axon and at the synapse, voltage-gated ion channels directionally propagate electrical signals. Voltage-gated ion-channels are usually ion-specific, and channels specific to sodium (Na+), potassium (K+), calcium (Ca2+), and chloride (Cl−) ions have been identified. The opening and closing of the channels are triggered by changing ion concentration, and hence charge gradient, between the sides of the cell membrane. [Return to image.]
Figure 15.31 Image description: Leakage channels are the simplest type of ion channel, in that their permeability is more or less constant. The types of leakage channels with the greatest significance in neurons are potassium and chloride channels. Although they are the simplest in theory, most conduct better in one direction than the other (they are rectifiers), and some are capable of being shut off by ligands even though they do not require ligands in order to operate. [Return to image.]
Figure 15.32 Image description: Measuring Charge across a Membrane with a Voltmeter. A recording electrode is inserted into the cell, and a reference electrode is outside the cell. By comparing the charge measured by these two electrodes, the transmembrane voltage is determined. It is conventional to express that value for the cytosol relative to the outside. [Return to image.]
Figure 15.33 Image description: What has been described here is the action potential, which is presented as a graph of voltage over time. It is the electrical signal that nervous tissue generates for communication. The change in the membrane voltage from −70 mV at rest to +30 mV at the end of depolarization is a 100-mV change. That can also be written as a 0.1-V change. To put that value in perspective, think about a battery. An AA battery that you might find in a television remote has a voltage of 1.5 V, or a 9-V battery (the rectangular battery with two posts on one end) is, obviously, 9 V. The change seen in the action potential is one or two orders of magnitude less than the charge in these batteries. In fact, the membrane potential can be described as a battery. A charge is stored across the membrane that can be released under the correct conditions. A battery in your remote has stored a charge that is “released” when you push a button. [Return to image.]
Figure 15.34 Image description: An action potential has three phases: depolarization, overshoot, repolarization. There are two more states of the membrane potential related to the action potential. The first one is hypopolarization, which precedes the depolarization, while the second one is hyperpolarization, which follows the repolarization. [Return to image.]
Figure 15.35 Image description: Action potentials in neurons that lack myelin sheaths travel much more slowly than action potentials in equivalent neurons sheathed in myelin. The speed of action potentials is also dependent on the diameter of the axon. Wider axons have lower resistance than narrow axons, and signals can travel faster in large axons. [Return to image.]
Figure 15.36 Image description: When myelination is present, the action potential propagates differently. Sodium ions that enter the cell at the initial segment start to spread along the length of the axon segment, but there are no voltage-gated Na+ channels until the first node of Ranvier. Because there is not a constant opening of these channels along the axon segment, the depolarization spreads at an optimal speed. The distance between nodes is the optimal distance to keep the membrane still depolarized above threshold at the next node. As Na+ spreads along the inside of the membrane of the axon segment, the charge starts to dissipate. If the nodes were any farther down the axon, that depolarization would have fallen off too much for voltage-gated Na+ channels to be activated at the next node of Ranvier. If the nodes were any closer together, the speed of propagation would be slower. [Return to image.]
Figure 15.37 image description: Communication at a chemical synapse: Communication at chemical synapses requires the release of neurotransmitters. When the presynaptic membrane is depolarized, voltage-gated Ca2+ channels open and allow Ca2+ to enter the cell. The calcium entry causes synaptic vesicles to fuse with the membrane and release neurotransmitter molecules into the synaptic cleft. The neurotransmitter diffuses across the synaptic cleft and binds to ligand-gated ion channels in the postsynaptic membrane, resulting in a localized depolarization or hyperpolarization of the postsynaptic neuron. [Return to image.]
Cord-like bundle of axons located in the peripheral nervous system that transmits sensory input and response output to and from the central nervous system.
Supportive neural cells.
Excitable neural cell that transfer nerve impulses.
In neurons, that portion of the cell that contains the nucleus; the cell body, as opposed to the cell processes (axons and dendrites).
In cells, an extension of a cell body; in the case of neurons, this includes the axon and dendrites.
Single process of the neuron that carries an electrical signal (action potential) away from the cell body toward a target cell.
One of many branchlike processes that extends from the neuron cell body and functions as a contact for incoming signals (synapses) from other neurons or sensory cells.
Regions of the nervous system containing cell bodies of neurons with few or no myelinated axons; actually may be more pink or tan in color but called gray in contrast to white matter.
Regions of the nervous system containing mostly myelinated axons, making the tissue appear white because of the high lipid content of myelin.
Lipid-rich insulating substance surrounding the axons of many neurons, allowing for faster transmission of electrical signals.
(In nervous system) a localized collection of neuron cell bodies that are functionally related; a “center” of neural function (plural= nuclei).
Localized collection of neuron cell bodies in the peripheral nervous system.
Cord-like bundle of axons located in the peripheral nervous system that transmits sensory input and response output to and from the central nervous system.
Functional division of the nervous system that is concerned with conscious perception, voluntary movement, and skeletal muscle reflexes.
Functional division of the nervous system that is responsible for homeostatic reflexes that coordinate control of cardiac and smooth muscle, as well as glandular tissue.
Two or more atoms covalently bonded together.
A substance composed of two or more different elements joined by chemical bonds.
Atom with an overall positive or negative charge. Many function as electrolytes.
Type of sweat gland that is common throughout the skin surface; it produces a hypotonic sweat for thermoregulation.
Type of sweat gland that is associated with hair follicles in the armpits and genital regions.
(In physiology) under conscious control of the brain.
(In physiology) though under nervous control (usually from the brain), control is not conscious.
Steady state of body systems that living organisms maintain.
Neural tissue associated with the digestive system that is responsible for nervous control through autonomic connections.
In reference to cells, this central organelle contains most of the DNA and is important in regulating cellular activities. A separate connotation for nucleus relates to islands of brain tissue where nerve cell bodies are concentrated.
Narrow junction across which a chemical signal passes from neuron to the next, initiating a new electrical signal in the target cell.
Information flow in one direction.
Tapering of the neuron cell body that gives rise to the axon.
Supportive neural cells.
Single stretch of the axon insulated by myelin and bounded by nodes of Ranvier at either end (except for the first, which is after the initial segment, and the last, which is followed by the axon terminal).
End of the axon, where there are usually several branches extending toward the target cell.
Swelling at the end of an axon where neurotransmitter molecules are released onto a target cell across a synapse.
Narrow junction across which a chemical signal passes from neuron to the next, initiating a new electrical signal in the target cell.
Shape of a neuron that has multiple processes—the axon and two or more dendrites.
Region of the adult brain connected primarily to the pons that developed from the metencephalon (along with the pons) and is largely responsible for comparing information from the cerebrum with sensory feedback from the periphery through the spinal cord.
Glial cell type in the CNS that provides the myelin insulation for axons in tracts.
Glial cell type in the PNS that provides the myelin insulation for axons in nerves.
Single stretch of the axon insulated by myelin and bounded by nodes of Ranvier at either end (except for the first, which is after the initial segment, and the last, which is followed by the axon terminal).
Lipid-rich layer of insulation that surrounds an axon, formed by oligodendrocytes in the CNS and Schwann cells in the PNS; facilitates the transmission of electrical signals.
An amphipathic lipid molecule containing a phosphate head (polar) and two fatty acid tails (non-polar). The major molecule comprising plasma membranes.
Region of the adult brain that develops from the telencephalon and is responsible for higher neurological functions such as memory, emotion, and consciousness.
One half of the bilaterally symmetrical cerebrum.
Outer gray matter covering the forebrain, marked by wrinkles and folds known as gyri and sulci.
(In nervous system) a localized collection of neuron cell bodies that are functionally related; a “center” of neural function (plural= nuclei).
Mapping of regions of the cerebral cortex based on microscopic anatomy that relates specific areas to functional differences, as described by Brodmann in the early 1900s.
Nuclei of the cerebrum (with a few components in the upper brain stem and diencephalon) that are responsible for assessing cortical movement commands and comparing them with the general state of the individual through broad modulatory activity of dopamine neurons; largely related to motor functions, as evidenced through the symptoms of Parkinson’s and Huntington’s diseases.
An important neurotransmitter.
Region of the adult brain that retains its name from embryonic development and includes the thalamus and hypothalamus.
Referring to the sense of smell.
Portion of the ventricular system that is in the region of the diencephalon.
Major region of the diencephalon that is responsible for relaying information between the cerebrum and the hindbrain, spinal cord, and periphery.
Region of the diencephalon containing the pineal gland.
Nucleus within the basal nuclei that is part of the indirect pathway.
Region of the brain inferior to the thalamus that functions in neural and endocrine signaling, temperature regulation, and control of the autonomic nervous system.
Tissue or organ that secretes hormones into the blood and lymph without ducts such that they may be transported to organs distant from the site of secretion.
Structures at the edge (limit) of the boundary between the forebrain and hindbrain that are most associated with emotional behavior and memory formation.
Middle region of the adult brain that develops from the mesencephalon.
Posterior region of the adult brain that develops from the rhombencephalon and includes the pons, medulla oblongata, and cerebellum.
Portion of the brainstem connecting the medulla oblongata with the midbrain. Serves as a connection to cerebellum, as well as functions including sleep cycles and the origin of some cranial nerves.
Lowest (most inferior) part of the brain, controlling many autonomic functions including heart rate, breathing, and digestion.
Connection of the ventricular system between the third and fourth ventricles located in the midbrain.
Half of the midbrain tectum that is part of the brain stem auditory pathway.
Half of the midbrain tectum that is responsible for aligning visual, auditory, and somatosensory spatial perceptions.
Diffuse region of gray matter throughout the brain stem that regulates sleep, wakefulness, and states of consciousness.
Ridge formed by convolutions on the surface of the cerebrum or cerebellum.
Groove formed by convolutions in the surface of the cerebral cortex.
General anatomical term for a hole or opening (usually in bone. Plural = foramina).
Neck
Mid-back, where ribs attach to vertebrae.
Lower back, below the ribs.
Gray matter region of the spinal cord in which sensory input arrives, sometimes referred to as the dorsal horn.
Gray matter of the spinal cord containing multipolar motor neurons, sometimes referred to as the ventral horn.
Region of the spinal cord gray matter in the thoracic, upper lumbar, and sacral regions that is the central component of the sympathetic division of the autonomic nervous system.
Region of the sacrum, bone forming the back part of the pelvic cavity.
Branch of the autonomic nervous system associated with emergency systems (“fight or flight”).
Central nervous system fibers carrying sensory information from the spinal cord or periphery to the brain.
Central nervous system fibers carrying motor commands from the brain to the spinal cord or periphery.
Protective outer coverings of the CNS composed of connective tissue.
Tough, fibrous, outer layer of the meninges that is attached to the inner surface of the cranium and vertebral column and surrounds the entire CNS.
Middle layer of the meninges named for the spider-web–like trabeculae that extend between it and the pia mater.
Filaments between the arachnoid and pia mater within the subarachnoid space.
Thin, innermost membrane of the meninges that directly covers the surface of the CNS.
Extracellular fluid in the small spaces between cells not contained within blood vessels.
Remnants of the hollow center of the neural tube that are spaces for cerebrospinal fluid to circulate through the brain.
Circulatory medium within the CNS that is produced by ependymal cells in the choroid plexus filtering the blood.
Specialized structures containing ependymal cells lining blood capillaries that filter blood to produce CSF in the four ventricles of the brain.
Portions of the ventricular system that are in the region of the cerebrum.
The portion of the ventricular system that is in the region of the brain stem and opens into the subarachnoid space through the median and lateral apertures.
Lowest (most inferior) part of the brain, controlling many autonomic functions including heart rate, breathing, and digestion.
Space between the arachnoid mater and pia mater that contains CSF and the fibrous connections of the arachnoid trabeculae.
Glial cell type that filters blood at the choroid plexus.
A solution containing ions; sometimes referring to ions themselves.
Outpocket of the arachnoid membrane into the dural sinuses that allows for reabsorption of CSF into the blood.
Any of the venous structures surrounding the brain, enclosed within the dura mater, which drain blood from the CNS to the common venous return of the jugular veins.
One of a pair of major veins located in the neck region that flows parallel to the common carotid artery that is more or less its counterpart; primarily drains blood from the brain, receives the superficial facial vein, and empties into the subclavian vein.
Sensory ganglion attached to the posterior nerve root of a spinal nerve.
Type of tissue that serves to hold in place, connect, and integrate the body’s organs and systems.
One of twelve nerves connected to the brain that are responsible for sensory or motor functions of the head and neck.
Tenth cranial nerve; responsible for the autonomic control of organs in the thoracic and upper abdominal cavities.
Division of the anterior (ventral) cavity that houses the heart, lungs, esophagus, and trachea.
Usually attached to bone, under voluntary control, each cell is a fiber that is multinucleated and striated.
Circuit of a reflex that involves a sensory input and motor output, or an afferent branch and an efferent branch, and an integrating center to connect the two branches.
Four muscles that extend and stabilize the knee.
Change in voltage of a cell membrane in response to a stimulus that results in transmission of an electrical signal; unique to neurons and muscle fibres.
Muscle that opposes the action of an agonist.
Three long muscles on the back of the upper leg.
Two-headed muscle that crosses the shoulder and elbow joints to flex the forearm while assisting in supinating it and flexing the arm at the shoulder.
Three-headed muscle that extends the forearm.
Opposite side of the body.
One of six muscles originating out of the bones of the orbit and inserting into the surface of the eye, which are responsible for moving the eye.
Division of the autonomic nervous system responsible for restful and digestive functions.
Organ that can cause a change in a value.
Alternate name for the sympathetic division of the autonomic nervous system that is based on the anatomical location of central neurons in the lateral horn of the thoracic and upper lumbar spinal cord.
Specifically referring to the cell body of a neuron in the autonomic system that is located in the central nervous system, specifically the lateral horn of the spinal cord or a brain stem nucleus.
Series of ganglia adjacent to the vertebral column that receive input from central sympathetic neurons.
Axon from a central neuron in the autonomic nervous system that projects to and synapses with a ganglionic neuron; sometimes referred to as a preganglionic neuron.
Axon from a ganglionic neuron in the autonomic nervous system that projects to and synapses with the target effector; sometimes referred to as a postganglionic neuron.
Inner layer of the adrenal glands that plays an important role in the stress response by producing epinephrine and norepinephrine.
Endocrine glands located at the top of each kidney that are important for the regulation of the stress response, blood pressure and blood volume, water homeostasis, and electrolyte levels.
Paired nerves that carry both autonomic and sensory fibers to the internal organs.
Chemical signal that is released from the synaptic end bulb of a neuron to cause a change in the target cell.
Alternate name for the parasympathetic division of the autonomic nervous system that is based on the anatomical location of central neurons in brain-stem nuclei and the lateral horn of the sacral spinal cord; also referred to as craniosacral outflow.
Protein molecule that contains a binding site for another specific molecule (called a ligand).
Synapse at which acetylcholine is released and binds to the nicotinic or muscarinic receptor.
Synapse where norepinephrine is released, which binds to α- or β-adrenergic receptors.
Signaling molecule released as a neurotransmitter by most postganglionic sympathetic fibers as part of the sympathetic response or as a hormone into the bloodstream from the adrenal medulla.
Signaling molecule released from the adrenal medulla into the bloodstream as part of the sympathetic response.
Secretion of an endocrine organ that travels via the bloodstream or lymphatics to induce a response in target cells or tissues in another part of the body.
Sensory receptor specialized for temperature stimuli.
Change in the membrane potential that varies in size, depending on the size of the stimulus that elicits it.
Unit outline
Learning Objectives
At the end of this unit, you should be able to:
I. Identify and describe the components of the integumentary system.
II. Identify and describe the five layers of the epidermis of the skin, including the location and function of keratinocytes and melanocytes.
III. Specify the function(s) of epidermal derivatives, including hair, sebaceous glands, sudoriferous glands, ceruminous glands, and nails.
IV. Describe five major functions of the integumentary system.
V. Explain the origin of variation in pigmentation in skin and integumentary derivatives.
VI. Explain underlying causes of common integumentary system conditions.
What do you think of when you look at your skin in the mirror? Do you think about cleaning and caring for it, checking for damage from the sun, or maybe adding a tattoo? Or do you think about the fact that the skin is an organ belonging to one of the body’s most essential and dynamic systems, the integumentary system? The skin makes up about 16 percent of body weight of an adult and covers an area of 1.5 to 2 m2, making it the largest organ system in the human body.
The integumentary system refers to the skin, as well as accessory structures, such as hair, nails, and glands. The skin is responsible for much more than your outward appearance, beginning with acting as a protective covering between your internal and external environments. This chapter will introduce the structure and many other functions of the integumentary system.
Part 1: Layers of the Skin
Although you may not typically think of the skin as an organ, it is in fact comprised of different types of tissues that work together to perform unique and critical functions. The skin is comprised of two main layers: an outer epidermis and a deeper dermis (Figure 9.1). The deeper layer of skin is well vascularized (i.e., has numerous blood vessels). It also has numerous sensory and nerve fibers ensuring communication to and from the brain. The skin is ultimately affixed to deeper body structures, such as muscle, by connective tissue in a subcutaneous layer known as the hypodermis.
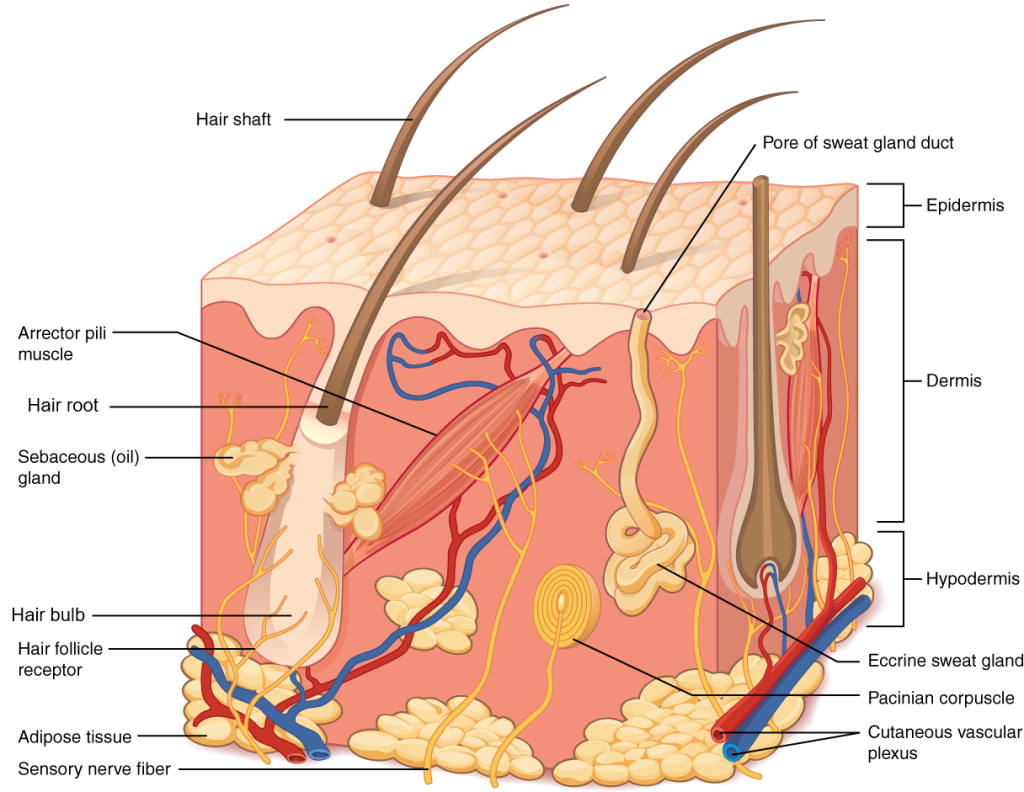
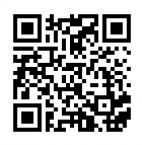
Epidermis
The epidermis (Figures 9.2 & 9.3) is composed of keratinized, stratified squamous epithelium. Like all epithelial tissue, it is avascular. The dark-staining epithelial cells of the epidermis are arranged in multiple strata with the outermost cells appearing the most squamous in shape. The majority of skin areas is classified as thin skin, meaning that has four visible layers(i.e., strata) of cells (Figure 9.2). From deepest to superficial, these layers are the stratum basale, stratum spinosum, stratum granulosum, and stratum corneum. Thick skin, however, is found exclusively on the palms of the hands and the soles of the feet. This type of epidermis has an additional fifth layer, called the stratum lucidum, located between the stratum corneum and the stratum granulosum (Figures 9.3 & 9.4).
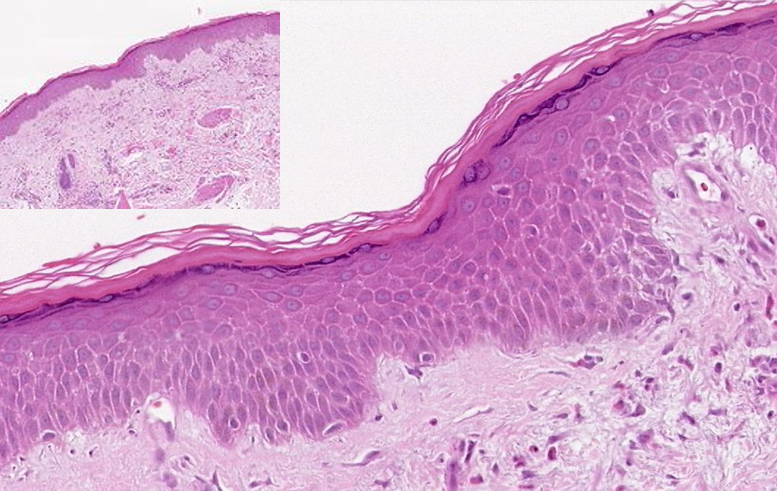
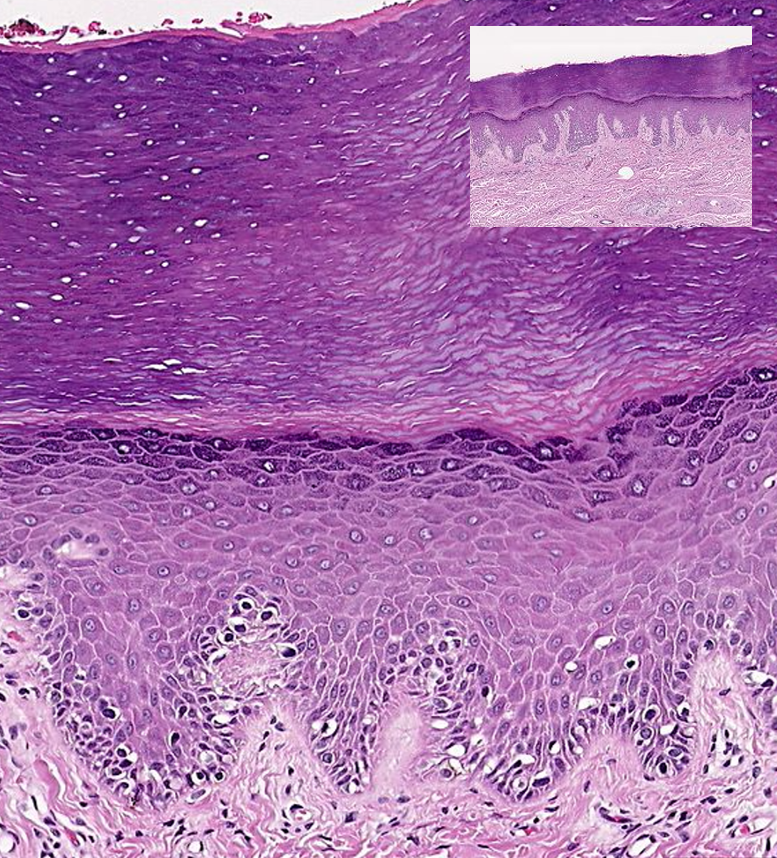
Regardless of whether skin is thick or thin, the dominant cells found in the epidermis are called keratinocytes. A keratinocyte is a cell that manufactures and stores the protein keratin. Keratin is an intracellular fibrous protein that confers hardness and water-resistance to skin, hair, and nails. Because the skin covers so much surface area, the presence of keratin helps prevent excessive water loss across the skin surface. The maturation of keratinocytes proceeds from the innermost towards the outermost layers. By the point the keratinocytes reach the stratum corneum they have died and regularly slough away but will be replaced continuously by cells originating from the deeper layers (Figure 9.4).
1. Stratum Basale: The stratum basale (also called the stratum germinativum) is the deepest epidermal layer and attaches the epidermis to the basal lamina, below which lie the layers of the dermis. The cells in the stratum basale bond to the dermis via intertwining collagen fibres that make up the basement membrane.
A finger-like projection, or fold, known as the dermal papilla (plural = dermal papillae) is found in the superficial portion of the dermis. Dermal papillae increase the strength of the connection between the epidermis and dermis; the greater the folding, the stronger the connection made (Figure 9.6). In a growing fetus, fingerprints form where the cells of the stratum basale meet the papillae of the underlying dermal layer (papillary layer), resulting in the formation of the ridges on your fingers that you recognize as fingerprints. Why these ridges form in the pattern that you see is a result of genetics and the fetal environment. Fingerprints are unique to each individual and are used for forensic analyses because the patterns do not change with the growth and aging processes.
The stratum basale is a single layer of cells primarily made of basal cells. A basal cell is a cuboidal-shaped stem cell that is a precursor of the keratinocytes of the epidermis. All of the keratinocytes are produced from this single layer of cells, which are constantly going through growth (mitosis) to produce new cells.
As new cells are formed, the existing cells are pushed superficially away from the stratum basale. Two other cell types are found dispersed among the basal cells in the stratum basale. The first is a Merkel cell, which functions as a receptor and is responsible for stimulating sensory nerves that the brain perceives as touch. These cells are especially abundant on the surfaces of the hands and feet. The second is a melanocyte, a cell that produces the pigment melanin. Melanin gives hair and skin its color, and also helps protect the living cells of the epidermis from ultraviolet (UV) radiation damage.
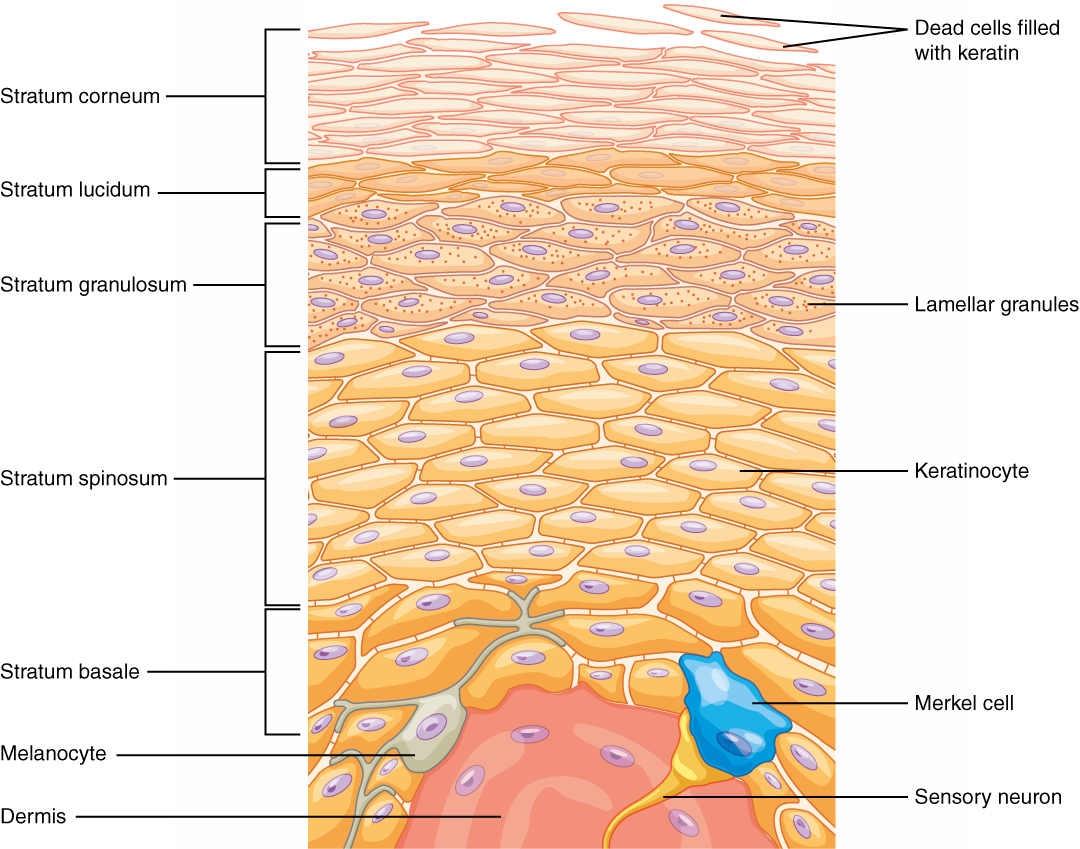
2. Stratum Spinosum: As the name suggests, the stratum spinosum is spiny in appearance due to the protruding cell processes that join the cells via a structure called a desmosome. The desmosomes interlock with each other and strengthen the bond between the cells. It is interesting to note that the “spiny” nature of this layer is an artifact of the staining process. Unstained epidermis samples do not exhibit this characteristic appearance. The stratum spinosum is composed of eight to 10 layers of keratinocytes, formed as a result of cell division in the stratum basale (Figure 9.4). Also Interspersed among the keratinocytes of this layer, but not shown in Figure 9.4, is a type of dendritic cell called the Langerhans cell, which functions as a macrophage by engulfing bacteria, foreign particles, and damaged cells that occur in this layer.
The keratinocytes in the stratum spinosum begin the synthesis of keratin and release a water-repelling glycolipid that helps prevent water loss from the body, making the skin relatively waterproof. As new keratinocytes are produced atop the stratum basale, the keratinocytes of the stratum spinosum are pushed into the stratum granulosum.
3. Stratum Granulosum: The stratum granulosum has a grainy appearance due to further changes to the keratinocytes as they are pushed from the stratum spinosum. The cells (three to five layers deep) become flatter, their cell membranes thicken, and they generate large amounts of the proteins keratin, which is fibrous, and keratohyalin, which accumulates as granules within the cells (Figure 9.4). These two proteins make up the bulk of the keratinocyte mass in the stratum granulosum and give the layer its grainy appearance. The nuclei and other cell organelles disintegrate as the cells die, leaving behind the keratin, keratohyalin, and cell membranes that will form the stratum lucidum, the stratum corneum, and the accessory structures of hair and nails.
4. Stratum Lucidum: The stratum lucidum is a smooth, seemingly translucent layer of the epidermis located just above the stratum granulosum and below the stratum corneum. This thin layer of cells is found only in the thick skin of the palms, soles, and digits. The keratinocytes that compose the stratum lucidum are dead and flattened (Figure 9.4). These cells are densely packed with eleiden, a clear substance derived from keratohyalin that gives these cells their transparent (i.e., lucid) appearance and provides a barrier to water.
5. Stratum Corneum: The stratum corneum is the most superficial layer of the epidermis and is the layer exposed to the outside environment (Figure 9.4). The increased keratinization (also called cornification) of the cells in this layer gives it its name. There are usually 15 to 30 layers of cells in the stratum corneum. This dry, dead layer helps prevent the penetration of microbes and the dehydration of underlying tissues, and provides a mechanical protection against abrasion for the more delicate, underlying layers. Cells in this layer are shed periodically and are replaced by cells pushed up from the stratum granulosum (or stratum lucidum in the case of the palms and soles of feet). The entire layer is replaced during a period of about 4 weeks. Cosmetic procedures, such as microdermabrasion, help remove some of the dry, upper layer and aim to keep the skin looking “fresh” and healthy.
Dermis
The dermis might be considered the “core” of the integumentary system (derma- = “skin”), as distinct from the epidermis (epi- = “upon” or “over”) and hypodermis (hypo- = “below”). It contains blood and lymph vessels, nerves, and other structures, such as hair follicles and sweat glands. The dermis is made of two layers of connective tissue that compose an interconnected mesh of elastin and collagenous fibers, produced by fibroblasts (Figure 9.5).
1. Papillary Layer: The papillary layer is made of loose, areolar connective tissue, which means the collagen and elastin fibers of this layer form a loose mesh. This superficial layer of the dermis projects into the stratum basale of the epidermis to form finger-like dermal papillae (Figure 9.5). Within the papillary layer are fibroblasts, a small number of fat cells (adipocytes), and an abundance of small blood vessels.
In addition, the papillary layer contains phagocytes, defensive cells that help fight bacteria or other infections that have breached the skin. This layer also contains lymphatic capillaries, nerve fibers, and touch receptors called the tactile (Meissner ) corpuscles (Figure 9.11).
2. Reticular Layer: Underlying the papillary layer is the much thicker reticular layer, composed of dense, irregular connective tissue. This layer is well vascularized and has a rich sensory and nerve supply. The reticular layer appears reticulated (net-like) due to a tight meshwork of fibers. Elastin fibers provide some elasticity to the skin, enabling movement. Collagen fibers provide structure and tensile strength, with strands of collagen extending into both the papillary layer and the hypodermis. In addition, collagen binds water to keep the skin hydrated.
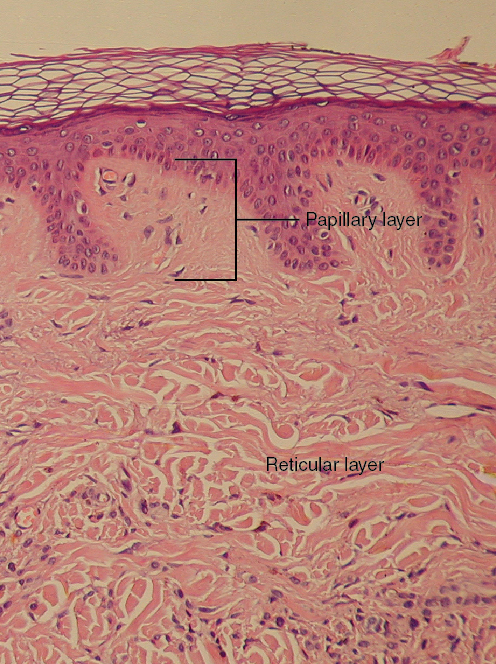
Hypodermis
The hypodermis (also called the subcutaneous layer or superficial fascia) is a layer directly below the dermis and serves to connect the skin to the underlying fascia (fibrous tissue) of the bones and muscles. It is not considered a part of the skin, although the border between the hypodermis and dermis can be difficult to distinguish. The hypodermis consists of well-vascularized, loose, areolar connective tissue and adipose tissue, which functions as a mode of fat storage and provides insulation and cushioning for the integument.
The hypodermis is one of the common injection sites for delivering medication because this layer contains relatively few blood vessels versus adipose tissue. Thus, medicines will be slowly absorbed by the body compared to other injection sites, such as a muscle or vein. Another term for an injection into the hypodermis is subcutaneous injection (Fugure 9.6). For example, insulin injections are administered subcutaneously.
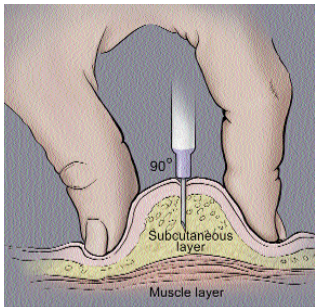
A correctly administered hypodermic injection places the needle into the skin at a 45-90 degree angle until the needle is completely covered by skin. No blood should appear in the syringe; if so, the needle should be repositioned before completing the injection.
Test Your Knowledge
I. Identify and describe the components of the integumentary system.
- List and identify all the organs and accessory structures of the integumentary system.
- Define “organ”. Explain why the skin specifically would fit your definition of an organ.
- For the two layers of the dermis, state their proper anatomical name and the specific tissue type of which each is primarily composed.
- Identify the accessory structures of the skin that are embedded in each of the two layers of the dermis.
- Draw an annotated diagram of the skin, clearly showing:
- The epidermal and dermal layers of the skin, and the hypodermis
- The type(s) of tissue of that comprise each of the above layers indicated above (hint: refer back to the Tissue Structure topic)
- The layer(s) of the skin that contain(s) blood vessels
- The layer(s) of the skin that contain(s) nervous structures
II. Identify and describe the five layers of the epidermis of the skin, including the location and function of keratinocytes and melanocytes.
- Draw an annotated diagram of the epidermis of thick skin, clearly showing the five layers of the epidermis and specifying the distinguishing physical characteristics of each layer.
- Identify which layer in the diagram you created above would be missing from thin skin.
- Specify the two main types of cells found in the epidermis, and which important chemical each of them produce. Briefly explain how each of those chemicals provide protection to underlying tissues.
Part 2: Accessory Structures of the Skin
Accessory structures of the skin include hair, nails, sweat glands, and sebaceous glands. These structures embryologically originate from the epidermis and can extend down through the dermis into the hypodermis.
Hair
Hair is a keratinous filament growing out of the epidermis. It is primarily made of dead, keratinized cells. Strands of hair originate in an epidermal penetration of the dermis called the hair follicle (Figures 9.7 & 9.8).
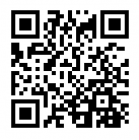
The hair shaft is the part of the hair not anchored to the follicle, and much of this is exposed at the skin’s surface. The rest of the hair, which is anchored in the follicle, lies below the surface of the skin and is referred to as the hair root. The hair root ends deep in the dermis at the hair bulb (which is the deep end of the hair follicle). The root includes a layer of actively growing basal cells called the hair matrix. The hair bulb surrounds the hair papilla, which is made of connective tissue and contains blood capillaries and nerve endings from the dermis (Figure 9.7).
Just as the basal layer of the epidermis forms the layers of epidermis that get pushed to the surface as the dead skin on the surface sheds, the basal cells of the hair bulb divide and push cells outward in the hair root and shaft. The external hair is completely dead and composed entirely of keratin. For this reason, hair does not have sensation. Furthermore, you can cut your hair or shave without damaging the hair structure because the cut is superficial. Most chemical hair removers also act superficially; however, electrolysis and plucking both attempt to destroy the hair bulb so hair cannot grow.
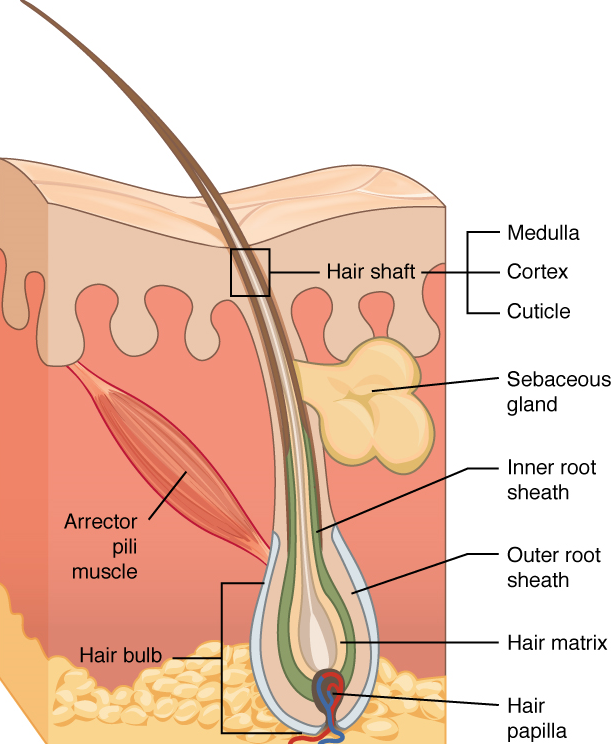
Hair serves a variety of functions in the human body, including protection, sensory input, and communication. For example, hair on the head protects the skull from the sun. The hair in the nose and ears, and around the eyes (eyelashes) defends the body by trapping and excluding dust particles that may contain allergens and microbes. Hair of the eyebrows prevents sweat and other particles from dripping into and bothering the eyes. Hair also has a sensory function due to sensory innervation by a hair root plexus surrounding the base of each hair follicle.
Hair is extremely sensitive to air movement or other disturbances in the environment, much more so than the skin surface. This feature is also useful for the detection of the presence of insects or other potentially damaging substances on the skin surface. Each hair root is connected to a smooth muscle called the arrector pili that contracts in response to nerve signals from the nervous system, making the external hair shaft “stand up” (Figure 9.7). The primary purpose for this would be to trap a layer of air to add insulation, but in humans the hairs are too far away from each other to be effective as insulation. The erection of the hair shafts in response to low body temperature nevertheless persists in humans and is visible as "goose bumps".
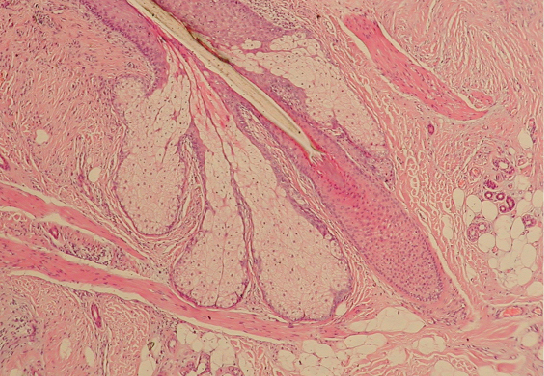
Hair grows and is eventually shed and replaced by new hair. On average, 50 hairs are lost and replaced per day. Hair loss occurs if there is more hair shed than what is replaced and can happen due to hormonal or dietary changes. Hair loss can also result from the aging process, or the influence of hormones.
Nails
The nail bed is a specialized structure of the epidermis that is found at the tips of our fingers and toes. The nail body is formed on the nail bed, and protects the tips of our fingers and toes as they are the farthest extremities and the parts of the body that experience the maximum mechanical stress (Figure 9.9). In addition, the nail body forms a back-support for picking up small objects with the fingers. The nail body is composed of densely packed dead keratinocytes. The nail body forms at the nail root, which has a matrix of proliferating cells from the stratum basale that enables the nail to grow continuously.
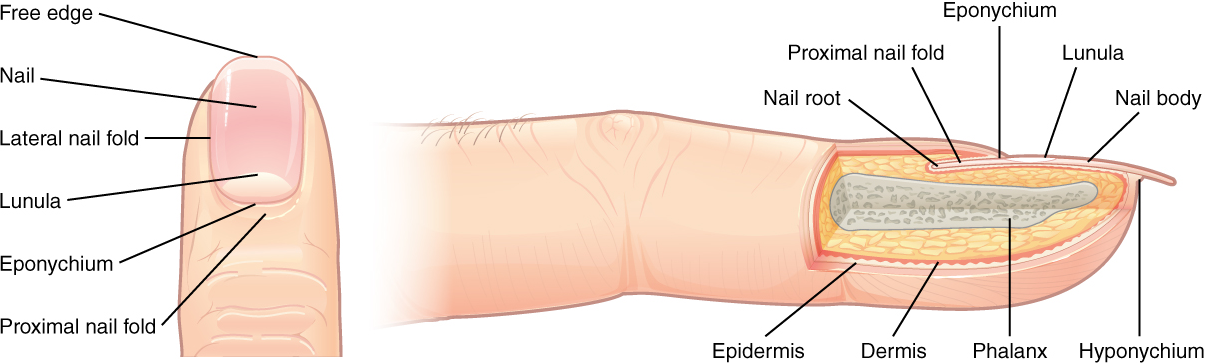
Sweat Glands
When the body becomes warm, sudoriferous glands produce sweat to cool the body. Sweat glands develop from epidermal projections into the dermis and are classified as merocrine glands; that is, the secretions are excreted by exocytosis through a duct without affecting the cells of the gland. There are two main types of sweat glands, each secreting slightly different products.
An eccrine sweat gland is type of gland that produces a hypotonic (relative to blood plasma) sweat for thermoregulation. These glands are found all over the skin’s surface, but are especially abundant on the palms of the hand, the soles of the feet, and the forehead (Figure 9.10).
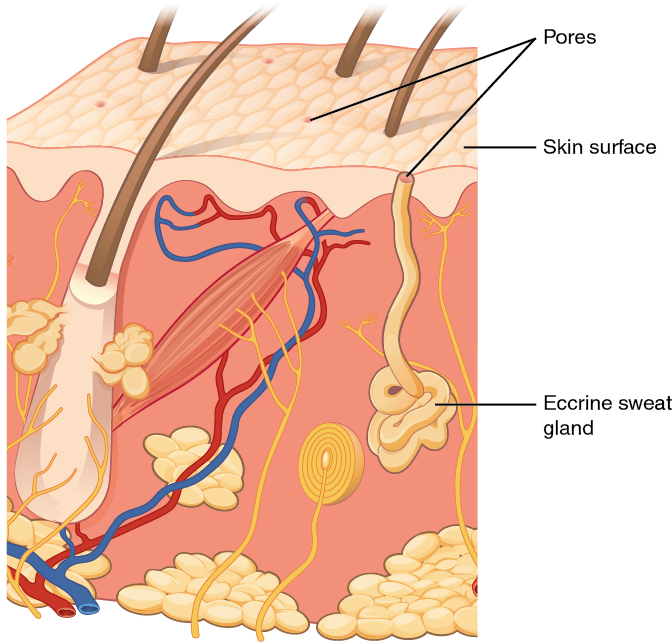
They are coiled glands lying deep in the dermis, with the duct rising up to a pore on the skin surface, where the sweat is released. This type of sweat, released by exocytosis, is composed mostly of water, with some salt, antibodies, traces of metabolic waste, and dermicidin, an antimicrobial peptide. Eccrine glands are a primary component of thermoregulation in humans and thus help to maintain homeostasis.
An apocrine sweat gland is usually associated with hair follicles in densely hairy areas, such as the armpits and anogenital regions. Apocrine sweat glands are larger than eccrine sweat glands and lie deeper in the dermis, sometimes even reaching the hypodermis, with the duct normally emptying into the hair follicle. In addition to water and salts, apocrine sweat includes organic compounds that make the sweat thicker and subject to bacterial decomposition and subsequent smell. The release of this sweat is under both nervous and hormonal control, and plays a role in the poorly understood human pheromone response. Most commercial antiperspirants use an aluminum-based compound as their primary active ingredient to stop sweat. When the antiperspirant enters the sweat gland duct, the aluminum-based compounds precipitate due to a change in pH and form a physical block in the duct, which prevents sweat from coming out of the pore.
There are several different types of modified apocrine glands that have become specialized to serve particular functions. One example is the mammary gland, which allows mammals to produces and secretes milk (a mixture of water, salt, and organic compounds) in appropriate concentrations to nourish growing offspring. Another example is the ceruminous gland, which is found in the external auditory meatus (ear canal) and secretes a pigmented mixture of lipids and proteins that combines with the sebum secreted from sebaceous glands (see below) and dead keratinocytes to form cerumen (earwax). This sticky substance is used to trap small foreign bodies (e.g. dirt, small insects) and help prevent damage to the tympanic membrane (eardrum).
Sebaceous Glands
A sebaceous gland is a type of oil gland that is found all over the body and helps to lubricate and waterproof the skin and hair. Most sebaceous glands are associated with hair follicles (Figures 9.7 & 9.8). They generate and excrete sebum, a mixture of lipids, onto the skin surface, thereby naturally lubricating the dry and dead layer of keratinized cells of the stratum corneum, keeping it pliable. The fatty acids of sebum also have antibacterial properties, and prevent water loss from the skin in low-humidity environments. The secretion of sebum is stimulated by hormones, many of which do not become active until puberty. Thus, sebaceous glands are relatively inactive during childhood.
Test Your Knowledge
III. Specify the function(s) of epidermal derivatives, including hair, sebaceous glands, sudoriferous glands, ceruminous glands, and nails.
- List the main function(s) served in human by the hairs of the:
- Scalp
- Eyelids
- Nostrils
- Rest of the body
- What is the main function(s) of nails, and what about their structure allows them to perform that function(s)?
- Complete the following table regarding integumentary glands:
Gland name | Location(s) in the body | Product contents | Product function(s) |
Eccrine sudoriferous gland | |||
Apocrine sudoriferous gland | |||
Ceruminous gland | |||
Sebaceous gland |
Part 3: Functions of the Integumentary System
The skin and accessory structures perform a variety of essential functions, such as protecting the body from invasion by microorganisms, chemicals, and other environmental factors; preventing dehydration; acting as a sensory organ; modulating body temperature and electrolyte balance; and synthesizing vitamin D. The underlying hypodermis has important roles in storing fats, forming a “cushion” over underlying structures, and providing insulation from cold temperatures.
Protection: The skin protects the rest of the body from the basic elements of nature such as wind, water, and UV sunlight. It acts as a protective barrier against water loss, due to the presence of layers of keratin and glycolipids in the stratum corneum. It also is the first line of defense against abrasive activity due to contact with grit, microbes, or harmful chemicals. Sweat excreted from sweat glands deters microbes from over-colonizing the skin surface by generating dermicidin, which has antibiotic properties.
Sensation: The fact that you can feel an ant crawling on your skin, allowing you to flick it off before it bites, is because the skin, and especially the hairs projecting from hair follicles in the skin, can sense changes in the environment. The hair root plexus surrounding the base of the hair follicle senses a disturbance, and then transmits the information to the central nervous system (brain and spinal cord), which can then respond by activating the skeletal muscles of your eyes to see the ant and the skeletal muscles of the body to act against the ant.
The skin acts as a sense organ because the epidermis, dermis, and the hypodermis contain specialized sensory nerve structures that detect touch, surface temperature, and pain. These receptors are more concentrated on the tips of the fingers, which are most sensitive to touch, especially the tactile (Meissner) corpuscle (Figure 9.11), which responds to light touch, and the lamellated (Pacinian) corpuscle, which responds to vibration.
Merkel cells (Figure 9.4), seen scattered in the stratum basale, are also touch receptors. In addition to these specialized receptors, there are sensory nerves connected to each hair follicle, pain and temperature receptors scattered throughout the skin, and motor nerves innervate the arrector pili muscles and glands. This rich innervation helps us sense our environment and react accordingly.
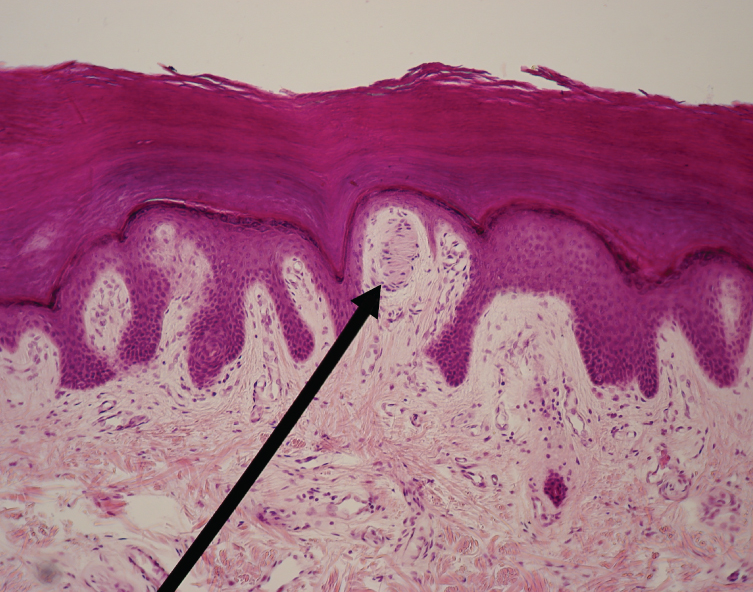
Thermoregulation: The integumentary system helps regulate body temperature through its tight association with the nervous system. The nervous system is continuously monitoring body temperature and initiating appropriate motor responses. Recall that sweat glands, accessory structures to the skin, secrete water, salt, and other substances to cool the body when it becomes warm.
Even when the body does not appear to be noticeably sweating, approximately 500 mL of sweat (insensible perspiration) are secreted a day. If the body becomes excessively warm due to high temperatures, vigorous activity (Figure 9.12ac), or a combination of the two, sweat glands will be stimulated by the sympathetic nervous system to produce large amounts of sweat, as much as 0.7 to 1.5 L per hour for an active person. When the sweat evaporates from the skin surface, the body is cooled as body heat is dissipated.
In addition to sweating, arterioles in the dermis dilate so that excess heat carried by the blood can dissipate through the skin and into the surrounding environment (Figure 9.12b). This accounts for the skin redness that many people experience when exercising.
When body temperatures drop, the arterioles constrict to minimize heat loss, particularly in the ends of the digits and tip of the nose. This reduced circulation can result in the skin taking on a whitish hue. Although the temperature of the skin drops as a result, passive heat loss is prevented, and internal organs and structures remain warm. If the temperature of the skin drops too much (such as environmental temperatures below freezing), the conservation of body core heat can result in the skin actually freezing, a condition called frostbite.
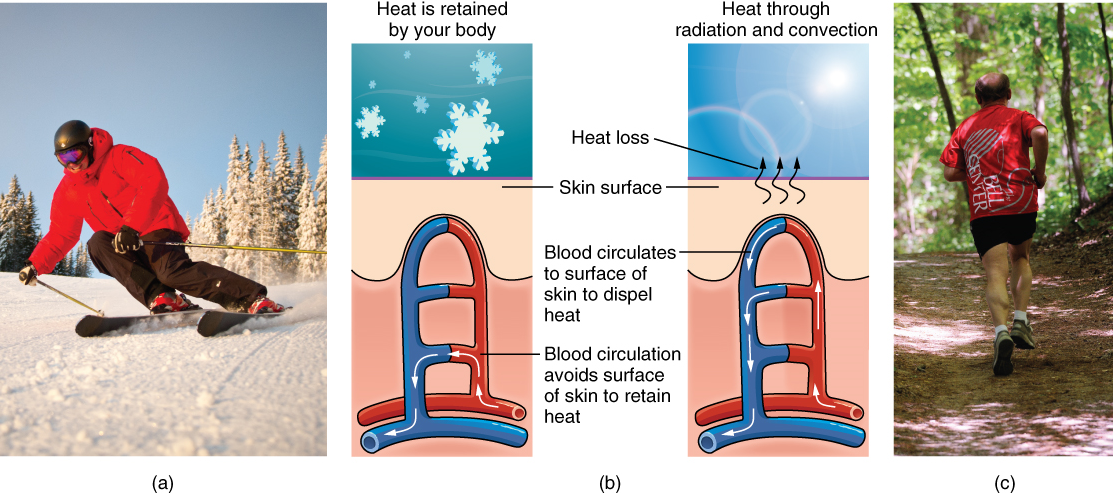
Other Functions: The epidermal layer of human skin synthesizes vitamin D when exposed to UV radiation. In the presence of sunlight, a precursor of vitamin D is synthesized from a derivative of the cholesterol in the skin. Further biochemical reactions in the liver and kidney produce then calcitriol, the active chemical form of the vitamin, which has important roles in the absorption of calcium from the small intestine In present day society, vitamin D is added as a supplement to many foods, including milk and orange juice, compensating for the need for sun exposure.
The skin is also a minor component of the excretory system, which is used to remove metabolic waste products from the body. Most metabolic waste products are removed from the body via the urinary and respiratory systems. However, the skin does release in sweat some of the metabolic waste products that are found in blood plasma, albeit at relatively low concentrations.
Test Your Knowledge
IV. Describe five major functions of the integumentary system.
- Explain the mechanisms by which the integumentary system carries out each of the following functions:
- Protection
- Body temperature regulation
- Sensation
- Synthesis of vitamin D
- Excretion
Part 4: Pigmentation
Most cells in the body are naturally colorless but the integumentary system appears colorful due in part to the presence of pigments. The colour of skin is influenced by a number of pigments, including melanin, carotene, and hemoglobin. Recall that melanin is produced by cells called melanocytes, which are found scattered throughout the stratum basale of the epidermis. The melanin is transferred in the keratinocytes via a cellular vesicle called a melanosome (Figure 9.13).
Dark-skinned individuals produce more melanin than those with pale skin. Exposure to the UV rays of the sun or a tanning salon causes melanin to be manufactured and built up in keratinocytes, as sun exposure stimulates keratinocytes to secrete chemicals that stimulate melanocytes. The accumulation of melanin in keratinocytes results in the darkening of the skin, or a tan. This increased melanin accumulation protects the DNA of epidermal cells from UV ray damage. In contrast, too much melanin can interfere with the production of vitamin D, an important nutrient involved in calcium absorption.
Figure 9.13. Skin Pigmentation. The relative coloration of the skin depends of the amount of melanin produced by melanocytes in the stratum basale and taken up by keratinocytes in the outer layers. [Image description.]
It requires about 10 days after initial sun exposure for melanin synthesis to peak, which is why pale-skinned individuals tend to suffer sunburns of the epidermis initially. Dark-skinned individuals can also get sunburns, but are more protected than are pale-skinned individuals. Melanosomes are temporary structures that are eventually destroyed by fusion with lysosomes; this fact, along with melanin-filled keratinocytes in the stratum corneum sloughing off, makes tanning impermanent.
Too much sun exposure can eventually lead to wrinkling due to the destruction of the cellular structure of the skin, and in severe cases, can cause sufficient DNA damage to result in skin cancer.
Persons with albinism do not produce any melanin (Figure 9.14) and their skin and integumentary derivatives, such as a hair, are devoid of pigmentation; this necessitates seeking enhanced protection against UV damage when out in the sunlight. This condition is recessively inherited and does not cause ill health effects other than vision. The lack of pigmentation throughout the eye, not just in the iris, can affect visual acuity,
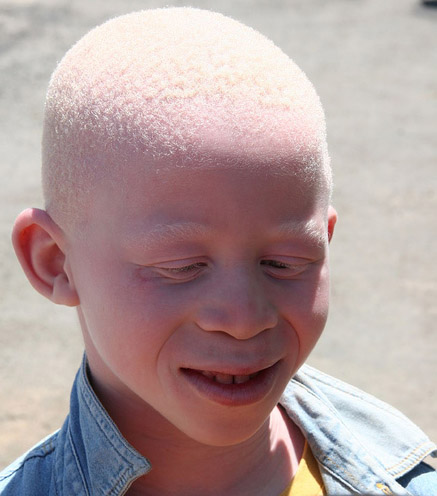
Patches of melanin-free skin characterizes the condition known as vitiligo (Figure 9.15). The primary cause is an autoimmune response that destroys the melanocytes in localized regions of the skin in the body but, unlike albinism, does not affect the whole skin surface of the body. This condition is more noticeable in dark-skinned individuals because the melanin-free patches stand out more. There are no additional health effects of having vitiligo and, moreover, it is not contagious.
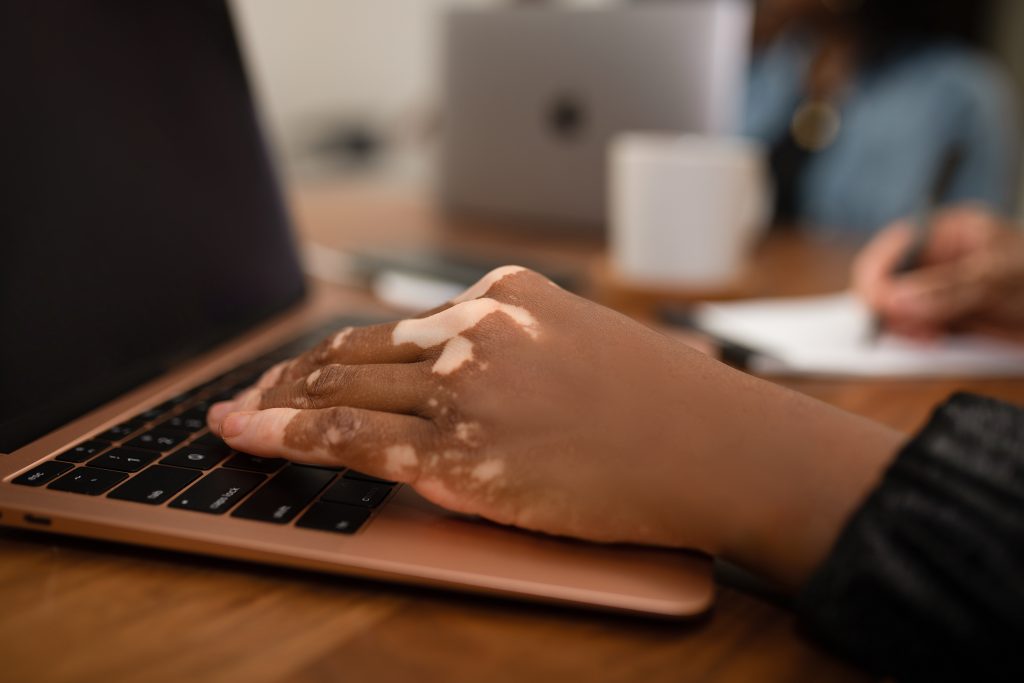
In addition to natural pigmentation, some persons augment skin color artificially by getting a tattoo. A permanent tattoo involves injecting artificial pigments into the dermal layer of the skin where it can be seen through the overlying epidermis. Some tattoo ink colors, however, do fade over time as the pigments are broken down by immune system cells and dispersed from the original pattern.
Variation in Skin Color
One of the most variable aspects of human anatomy is skin tone, resulting in a rainbow of color shades ranging from yellow to reddish to tan to brown to black. These different colors result from varying ratios of the dominant pigments of the skin: melanin and carotene.
While the number of melanocytes in the epidermis is the same among persons of different skin tones, the color differences result from differences in activity of cells and location of pigment deposition. For example. dark-skinned individuals have more active melanocytes than light-skinned individuals and dark-skinned individuals accumulate pigment in the more superficial stratum granulosum layer.
In addition, skin color is affected by blood flow through capillaries in the dermis which contributed red coloration from hemoglobin in red blood cells. Emotional states, especially in light-skinned individuals can be revealed by skin tone appearance. Skin can flush red during embarrassment or blanch white from fear. Indeed, the appearance of skin is noticeably different after death due to lack of oxygen and reduced blood circulation.
Excess blood pooling in the dermis of the skin, called a hematoma, due to damage from a punch or bump, is visible as a bruise through the outer layers of the skin. While initially red, the bruise color will gradually progress through a series of color changes --- blue/black to purple to green to yellow as hemoglobin is broken down and transformed into bilirubin.

Freckles and Moles
Dermatology is the medical specialty that studies the integument. In a dermatological exam, special attention is given to spots of pigmentation called freckles and moles. Freckles are flat spots of pigmentation that develop as a result of overactivity of melanocytes in these areas. They are prevalent in sun-exposed areas of the body, such as the face, arms, neck, and chest but will fade seasonally.
Moles (also known as nevi) are larger masses of melanocytes, and although most are benign, they should be monitored for changes that might indicate the presence of cancer (Figure 8). Cancerous moles may have an irregular border or uneven halves. In contrast to freckles, moles are raised and do not disappear with seasons.
Figure 9.16. Moles. Moles range from benign accumulations of melanocytes to melanomas. These structures populate the landscape of our skin. (credit: the National Cancer Institute) [Image description]
Hair color
Similar to the skin, hair gets its color from the pigment melanin, produced by melanocytes in the hair papilla. Different hair color results from differences in the type of melanin, which is genetically determined. As a person ages, the melanin production decreases, and hair tends to lose its color and becomes gray and/or white.
Test Your Knowledge
V. Explain the origin of variation in pigmentation in skin and integumentary derivatives.
- Describe how melanocytes transfer pigment to keratinocytes.
- Explain how skin tone differences are not related to melanocyte number.
- Describe the roles pigmentation and blood flow play in skin tone appearance.
- Use evolutionary past to explain dark-skinned and light-skinned individuals.
- Compare and contrast tanning, bruising, albinism, vitiligo, freckles, and moles in terms of origin, appearance, and longevity.
Part 5: Skin Conditions
Because the skin is the most conspicuous organ of the body, any changes to skin appearance may be noticeable to yourself and to others. Are these changes just due to variation among humans in skin color? Or do these changes indicate an underlying cause due to hormones, stress, or disease? Understanding these conditions can make you feel better about yourself and better informs how others see you. Your understanding of structure and function of the skin from previous sections of this chapter can be used to understand better these conditions.
Acne
Acne is a common skin condition that involves the appearance of temporary blemishes on the surface of the skin called pimples (Figure 9.17). These reddish and swollen structures can appear where hair follicles emerge from the skin surface. The association between acne and hair follicles results from a blockage of the oil (i.e., sebum) products from sebaceous glands. Normally these secretions lubricate the hair shaft that grows from the follicle and spill out to the surrounding epidermal surface. Sebum production increases during puberty; thus, teenagers often experience more outbreaks of acne than adults. However, pimples can develop throughout life and are often exacerbated by stress hormones.
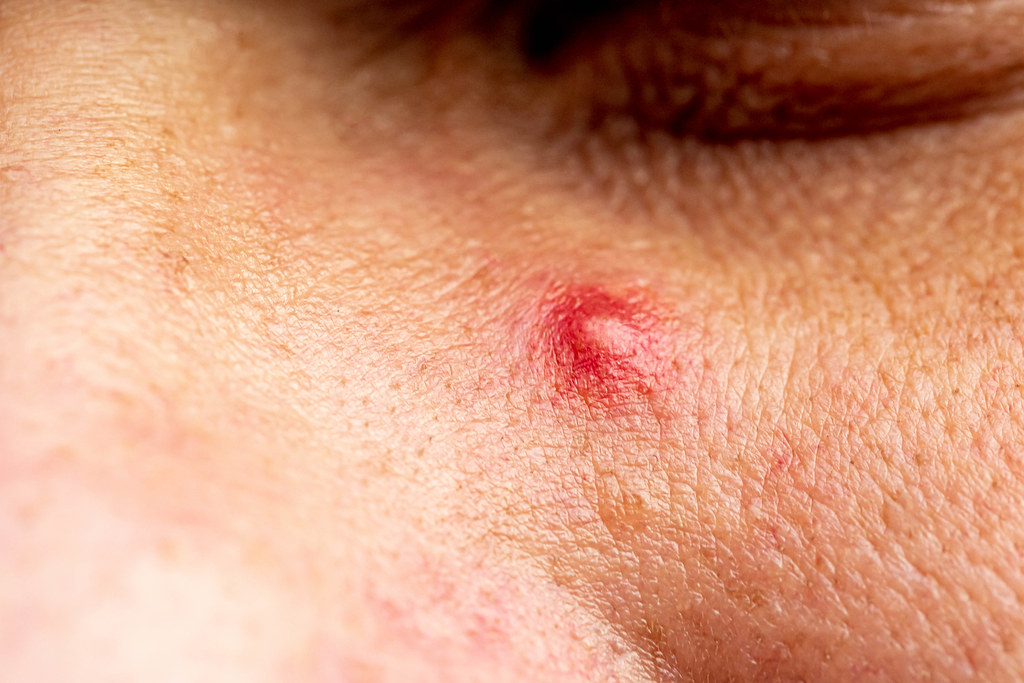
Burns
Burns are damage to skin causes by exposure to radiation, chemical, or electricity. Any burn is serious and warrants care to restore the important functions provided by the skin. But burn severity is typically ranked due to the extent of damage to the skin layers (Figure 9.18).
A first-degree burn, such as from a sun burn or brushing into a hot stove element, is the least severe category of burn severity. This kind of burn damage is limited to the epidermis, causes reddening, and is painful to the touch.
A second-degree burn involves both the epidermis and dermis. The burn damage extends into the dermal layer and may cause leakage of fluid, forming a blister (Figure 9.19). The loss of the epidermal protection and possible bursting of the blister makes the burn area more susceptible to infection. In addition, scars may appear in the healed area.
The third-degree burn is the most severe burn condition and involves the destruction of both the epidermal and dermal areas. The damaged region extends to the hypodermis and, because nerves are destroyed along with the dermis, painful sensation may be absent. This extensive burn damage cannot heal on its own and generally requires skin grafting.
Repair of burn damage requires both enhanced hydration and nutrition as there will be increased water loss across damaged areas and the regeneration of tissue is energetically expensive.

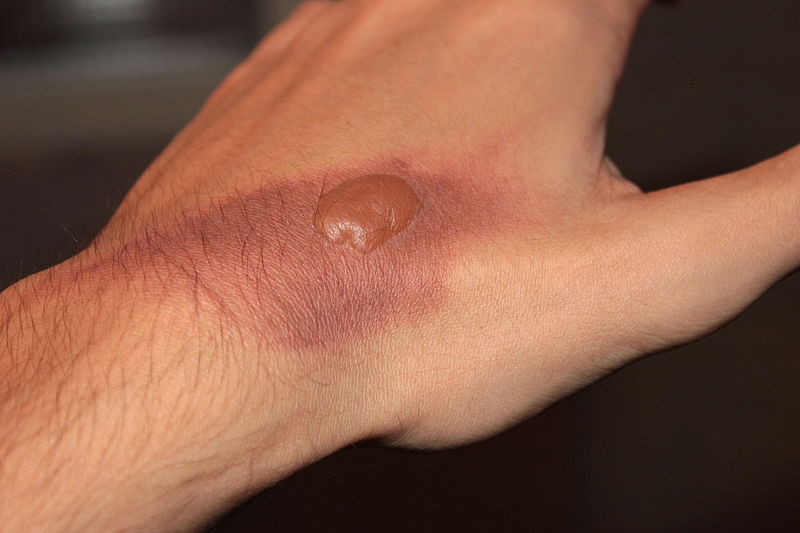
Eczema
Eczema, also known as atopic dermatitis, is an inflammation of the skin seen as a rash of eruptions (Figure 9.20) that vary in severity. These itchy irritated regions may become raw from scratching. Environmental conditions that stimulate eczema outbreaks include allergens, stress, soaps, fabrics. The condition is usually chronic but not contagious.

Hansen's Disease
Hansen's disease, also known as leprosy, is caused by a bacterial infection of Mycobacterium leprae (https://www.cdc.gov/leprosy/index.html). It affects not only the skin surface but also the nerves, eyes, and lining of the nasal passageway. The skin of infected persons develops thickened patches, swollen nodules, painless ulcers on different parts of the body, including the face, ears, and soles of the feet (Figure 9.21). Due to misinformation and prejudice, persons with Hansen's disease have been historically shunned. While the condition is mildly contagious, transmission would require months of close with an untreated infected person. The infection grows slowly and symptoms appear gradually but the condition is treatable with antibiotics. If left untreated however, the condition can develop into permanent paralysis, crippling of hands and feet, and disfigurement.
Louisiana has a special connection to Hansen's Disease by being the location of the National Leprosarium (https://www.hrsa.gov/hansens-disease/museum). This facility, located in Carville, a town about 18 miles southeast of Baton Rouge, operated for over 100 years from 1894 - 2005.
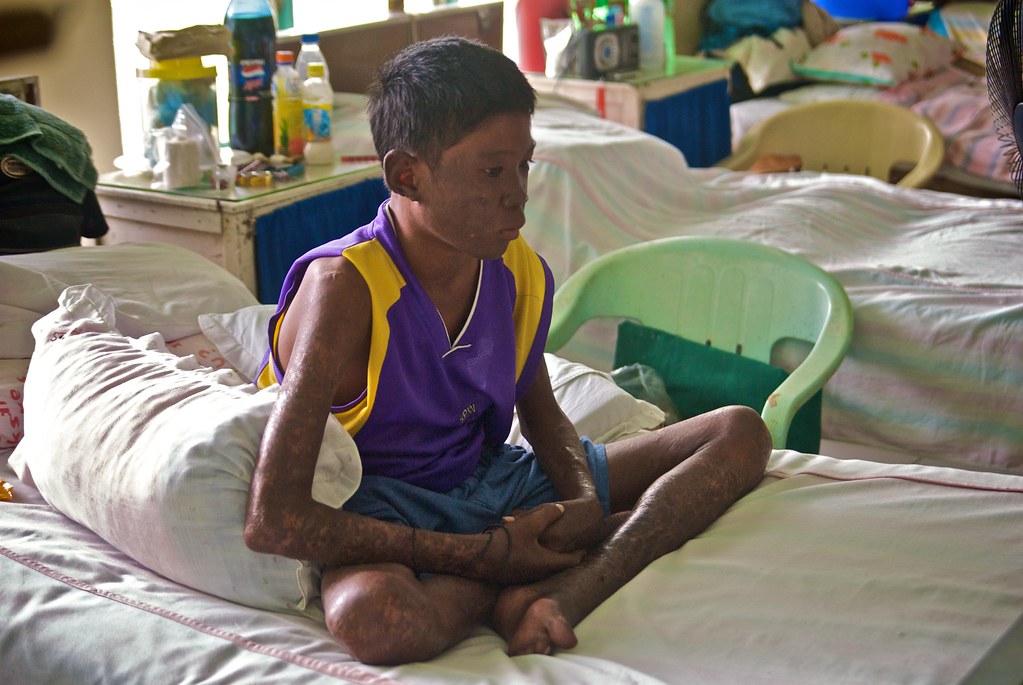
Psoriasis
Psoriasis is an autoimmune disorder that appears as regions of rash-like spots on the surface of some parts of the skin. Both eczema and psorias share common triggers for outbreaks but, unlike eczema, the rash is not an inflammation (i.e., dermatititis). Instead, psoriasis results from rapidly dividing skin cells that pile up on the skin surface of some body regions as well-defined scaly lesions (Figure 9.22). Persons with psoriasis do not experience as intense an itching sensation as those with eczema. and this condition is also not contagious.
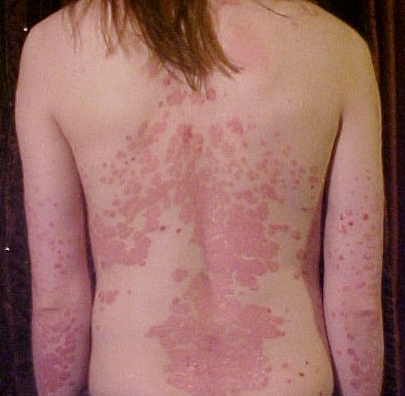
Test Your Knowledge
VI. Explain underlying causes of common integumentary system conditions.
- Define and explain the cause of each of the following integumentary system conditions:
-
- Acne
- Burns
- Eczema
- Hansen's disease
- Psoriasis
Practice
For the exercise below, drag the correct structure names to the empty boxes in the figure.
Image Descriptions
Figure 9.1 image description: This illustration shows a cross-section of skin tissue. The outermost layer is called the epidermis and occupies one-fifth of the cross-section. Several hairs are emerging from the surface. The epidermis dives around one of the hairs, forming a follicle. The middle layer is called the dermis, which occupies four-fifths of the cross-section. The dermis contains an erector pili muscle connected to one of the follicles. The dermis also contains an eccrine sweat gland, composed of a bunch of tubules. One tubule travels up from the bunch, through the epidermis, opening onto the surface a pore. There are two string-like nerves traveling vertically through the dermis. The right nerve is attached to a Pacinian corpuscle, a yellow structure consisting of concentric ovals similar to an onion. The lowest level of the skin, the hypodermis, contains fatty tissue, arteries, and veins. Blood vessels travel from the hypodermis and connect to hair follicles and erector pili muscle in the dermis.[Return to image.]
Figure 9.3 image description: A skin section viewed under a microscope that shows extensive outer layers of compressed keratinocytes in the epidermis. Even though the magnification used is the same as Figure 9.2 all layers are noticeably thicker and the underlying dermis is barely visible. [Return to image.]
Figure 9.4 image description: The bulk of the epidermis of the skin is comprised of layers (or strata) of stratified epithelial cells. The most common cell type is the keratinocyte but the appearance of the keratinocyte changes based on the strata. The lowermost layer, called the stratum basale, has a cell type called a melanocyte in addition to the keratinocyte. The melanocyte has tentacle-like extensions that reach up between the keratinocytes in the adjacent strata but the main part of the cell with the nucleus remains adjacent to the membrane separating the epidermis and dermis. The stratum spinosum layer is found above the stratum basale and the keratinocytes look different here because they have visible connections linking them together to adjacent cells. The next layer higher is called the stratum granulosum and the keratinocytes here are filled with granules. A change is cell shape is noteworthy as the strata layers accumulate; the outermost cells are flattened in appearance while the keratinocytes in the lower strata appear more cuboidal. The thinnest strata is the stratum lucidum that appears right above the stratum granulosum. The keratinocyte cells here still have their nuclei. In the final stratum, the outermost stratum corneum, the cells here are sloughing off from the epidermis and have lost their nuceli. [Return to image.]
Figure 9.5 image description: A skin section viewed under the microscope that shows in the interdigitation between the epidermis and dermis. Finger-like extensions of connective tissue in the papillary layer of the dermis project upwards into the epidermis. This resembles fingers from two different hands woven together. Below the extensions of the papillary layer, the bulk of the dermis is made up of connective tissue that is unorganized.[Return to image.]
Figure 9.6 image description: A syringe with needle is inserted into the skin at a ninety-degree angle. The skin has been pinched into a fold so that the fatty subcutaneous layer bulges for easier insertion. The needle is completely buried into the skin and only fat tissue cells are seen in the vicinity of the tip. Also shown is a muscular layer but this is located far below where the injection site occurs. [Return to image.]
Figure 9.7 image description: A cross section of the skin containing a hair follicle. The follicle is teardrop shaped. Its enlarged base, labeled the hair bulb, is embedded in the hypodermis. The outermost layer of the follicle is the epidermis, which invaginates from the skin surface to envelope the follicle. Within the epidermis is the outer root sheath, which is only present on the hair bulb. It does not extend up the shaft of the hair. Within the outer root sheath is the inner root sheath. The inner root sheath extends about half of the way up the hair shaft, ending midway through the dermis. The hair matrix is the innermost layer. The hair matrix surrounds the bottom of the hair shaft where it is embedded within the hair bulb. The hair shaft, in itself, contains three layers: the outermost cuticle, a middle layer called the cortex, and an innermost layer called the medulla. [Return to image.]
Figure 9.8 image description: A hair follicle is obvious in a skin section viewed under a microscope because it shows a finger-like projection of the epidermis plunging into the underlying dermis. The epidermal region is made up of more densely packed cells than the tissue in the dermis. The deepest part of the follicle is a rounded bulb of cells that appear to ensheath the hair. While these cells are solid in appearance, they are surrounded by clusters of sebaceous glandular cells that resemble inflated sacs. [Return to image.]
Figure 9.9 image description: The anatomy of the fingernail region. The left image shows a dorsal view of a finger. The proximal nail fold is the part underneath where the skin of the finger connects with the edge of the nail. The eponychium is a thin, pink layer between the white proximal edge of the nail (the lunula), and the edge of the finger skin. The lunula appears as a crescent-shaped white area at the proximal edge of the pink-shaded nail. The lateral nail folds are where the sides of the nail contact the finger skin. The distal edge of the nail is white and is called the free edge. An arrow indicates that the nail grows distally out from the proximal nail fold. The right image shows a lateral view of the nail bed anatomy. In this view, one can see how the edge of the nail is located just proximal to the nail fold. This end of the nail, from which the nail grows, is called the nail root. [Return to image.]
Figure 9.10 image description: An illustration of an eccrine sweat gland embedded in a cross section of skin tissue. The eccrine sweat gland is a bundle of white tubes embedded in the dermis. A single white tube travels up from the bundle and opens on to the surface of the epidermis. The opening is called a pore. There are several pores on the small block of skin portrayed in this diagram. [Return to image.]
Figure 9.11 image description: An arrow points to a cluster of nerve cells that resemble a bee hive. This bundle is located in a projection of the dermis up into the epidermis that causes it to be just underneath the skin's outer surface. Most of the dermal papillary layer projections do not contain this nervous structure. The nerve cells are distinctly clearer in appearance than the denser connective tissue cells in the surrounding dermis. [Return to image.]
Figure 9.12 image description: Part A is a photo of a man skiing with several snow-covered trees in the background. Part B is a diagram with a right and left half. The left half is titled “ Heat is retained by the body,” while the right half is titled “Heat loss through radiation and convection.” Both show blood flowing from an artery through three capillary beds within the skin. The beds are arranged vertically, with the topmost bed located along the boundary of the dermis and epidermis. The bottommost bed is located deep in the hypodermis. The middle bed is evenly spaced between the topmost and bottommost beds. In each bed, oxygenated blood (red) enters the bed on the left and deoxygenated blood (blue) leaves the bed on the right. The left diagram shows a picture of snowflakes above the capillary beds, indicating that the weather is cold. Blood is only flowing through the deepest of the three capillary beds, as the upper beds are closed off to reduce heat loss from the outer layers of the skin. The right diagram shows a picture of the sun above the capillary beds, indicating that the weather is hot. Blood is flowing through all three capillary beds, allowing heat to radiate out of the blood, increasing heat loss. Part C is a photo of a man running through a forested trail on a summer day. [Return to image.]
Figure 9.13 image description: A comparison of skin from dark (left) and light (right) skin tones shows skin color variants do not differ structurally. While the number of pigment-producing melanocytes in the stratum basale is the same, more pigment is ultimately produced by darker-skinned individuals. This is seen as relative more pigment-releasing vesicles budding off of the melanocytes and filling the keratinocytes with color. [Return to image.]
Figure 9.14 image description: A boy with albinism is seen smiling. His integument appears normal except that melanin is not produced, making the skin appear pinkish or pale. The lack of pigment does not just affect keratinocytes but is also seen where hair grows, such as eyebrows, eyelashes, and scalp. [Return to image.]
Figure 9.15 image description: A person with vitiligo is typing on a keyboard. Some fingers and parts of the palm shows patches of white skin on an otherwise dark-skinned individual. The patches are irregular shaped and not uniformly distributed on the integument; for example, the exposed wrist shows no vitiligo patches. [Return to image.]
Figure 9.16 image description: A series of images showing different appearances of non-malignant common moles on the skin. These small moles are roundish in shape with a regular edge and has even coloration of pink, tan, or black skin. Return to image.]
Figure 9.18 image description: A complex image of unburned skin (upper right) and burned skin (lower series). The unburned skin is comprised of undamaged epidermis, dermis, and hypodermis layers. In the First-degree burn image (lower left), redness indicates burn damage to the outer epidermis layers but the skin. The redness and damage extends to the entire epidermis and part of the underlying dermis in a Second-degree burn (lower middle). Finally, the most extensive damage and spread of redness is seen to all three skin layers; this is the Third-degree burn. The third-degree burned area is the thickest in damage and has completely burned through the epidermis and dermis layers, as well as penetrating substantially into the hypodermis region. [Return to image.]
Figure 9.20 image description: A diagram of a hand from a person with eczema shows rash-like irritations compared to surrounding areas of smooth skin. The irritation progresses through Redness (lower left), Blister (lower middle), and Flaking (lower right). The images convey a rawness to the rash that makes one think of wanting to scratch this irritation; itchiness is commonly associated with areas of the skin having an eczema outbreak.[Return to image.]
Figure 9.21 image description: A boy with Hansen's disease sits on a bed alone, not facing the camera. He has a pensive expression while waiting in a location that is presumably an institution, such as a hospital. The skin of his exposed arms and legs skin is covered extensively with scaly patches and crusty lesions. This condition has also affected his face, especially his cheek and the outside of his ears. [Return to image.]
Figure 9.22 image description: The back and arms of a person with psoriasis are covered with red patches, some of which appear as raised plaques. Although visibly red in contrast with the surrounding white skin, the condition looks dry without any blistering or flaking as in eczema. [Return to image.]
Synapse between the axon terminal of a motor neuron and the section of the membrane of a muscle fiber with receptors for the acetylcholine released by the terminal.
An amphipathic lipid molecule containing a phosphate head (polar) and two fatty acid tails (non-polar). The major molecule comprising plasma membranes.
“Water loving”; a molecule or portion thereof that is polar and therefore water soluble.
Membrane-spanning protein that has an inner pore, which allows the passage of one or more substances (a form of facilitated diffusion).
Form of transport across the cell membrane that requires input of cellular energy.
Difference in the concentration of a substance between two regions.
Membrane-spanning protein that binds to substances it needs to transport, changes shape, and moves the substance into or out of the cell (a form of facilitated diffusion or active transport pumps when energy is required).
Nucleotide containing ribose and an adenine base that is essential in energy transfer.
A channel protein (facilitated diffusion) that is activated (opens) when a molecule (such as a neurotransmitter) binds to it.
Ion channel protein (facilitated diffusion) that opens when a physical event directly affects the structure of the protein.
Sense of touch.
Ion channel that opens because of a change in the charge distributed across the membrane where it is located.
Ion channel (facilitated diffusion) that opens randomly and is not gated to a specific event, also known as a non-gated channel.
Distribution of charge across the cell membrane, based on the charges of ions.
Fluid outside cells (plasma or interstitial fluid).
Fluid inside cells.
Clear, semi-fluid medium of the cytoplasm, made up mostly of water.
Atom with a negative charge.
Chemical functional group, PO4-, a component of phospholipids and nucleic acids (including ATP).
The difference in voltage measured across a cell membrane under steady-state conditions, typically -70 mV.
Change in a cell membrane potential from rest toward zero.
Return of the membrane potential to its normally negative voltage at the end of the action potential.
Change in cell membrane potential below resting potential (<-70mV).
Time after the initiation of an action potential when another action potential cannot be generated.
Gap between two myelinated regions of an axon, allowing for strengthening of the electrical signal as it propagates down the axon.
Slow propagation of an action potential along an unmyelinated axon owing to voltage-gated Na+ channels located along the entire length of the cell membrane.
Quick propagation of the action potential along a myelinated axon owing to voltage-gated Na+ channels being present only at the nodes of Ranvier.
Membrane-bound structure that contains materials within or outside of the cell.
Export of a substance out of a cell by formation of a membrane-bound vesicle.
Small gap between cells in a chemical synapse where neurotransmitter diffuses from the presynaptic element to the postsynaptic element.
Movement of a substance from an area of higher concentration to one of lower concentration.
Molecule (usually a protein) that catalyzes chemical reactions.
Building block of proteins; characterized by an amino and carboxyl functional groups and a variable side-chain.
A type of covalent bond occurring between amino acids.
Ion with a positive charge.